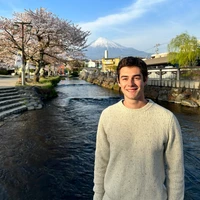
charlieoneill/embedding-saes
Updated
•
12
id
stringlengths 4
8
| author
sequencelengths 1
2.31k
⌀ | bibcode
stringlengths 19
19
| title
sequencelengths 1
2
| citation_count
int64 0
15.7k
| aff
sequencelengths 1
2.31k
⌀ | citation
sequencelengths 1
15.7k
⌀ | database
sequencelengths 1
4
| read_count
int64 0
645
| keyword
sequencelengths 1
58
| reference
sequencelengths 1
1.98k
⌀ | doi
sequencelengths 1
3
| subfolder
stringclasses 367
values | filename
stringlengths 13
25
| introduction
stringlengths 0
316k
| conclusions
stringlengths 0
229k
| year
int64 0
99
| month
int64 1
12
| arxiv_id
stringlengths 8
25
| abstract
stringlengths 1
9.28k
| failed_ids
bool 2
classes | keyword_search
sequencelengths 0
20
| umap_x
float32 -5.05
18.9
| umap_y
float32 -3.34
17.3
| clust_id
int64 -1
196
|
---|---|---|---|---|---|---|---|---|---|---|---|---|---|---|---|---|---|---|---|---|---|---|---|---|
12473133 | [
"Arca-Sedda, M.",
"Capuzzo-Dolcetta, R."
] | 2016MNRAS.461.4335A | [
"Globular clusters as tracers of the host galaxy mass distribution: the Fornax dSph test case"
] | 17 | [
"Department of Physics, Sapienza, University of Rome, Piazzale Aldo Moro 5, I-00185 Rome, Italy",
"Department of Physics, Sapienza, University of Rome, Piazzale Aldo Moro 5, I-00185 Rome, Italy"
] | [
"2017MNRAS.464.3060A",
"2017MNRAS.471..478A",
"2018A&A...615A..91B",
"2018MNRAS.477..480Z",
"2018MNRAS.477.4423A",
"2018MNRAS.479..900A",
"2018MNRAS.480..927P",
"2019ApJ...877..133D",
"2019MNRAS.485.2546B",
"2019MNRAS.488.2977O",
"2020MNRAS.493..320L",
"2020arXiv200906133A",
"2021MNRAS.503..594S",
"2021MNRAS.507.2339S",
"2022MNRAS.511.1860S",
"2022RMxAA..58..197A",
"2023MNRAS.523.2721H"
] | [
"astronomy"
] | 1 | [
"methods: numerical",
"galaxies: individual: Fornax",
"galaxies: nuclei",
"galaxies: star clusters: general",
"Astrophysics - Astrophysics of Galaxies"
] | [
"1911MNRAS..71..460P",
"1943ApJ....97..255C",
"1975PASJ...27..533M",
"1976ApJ...203...72T",
"1977MNRAS.181..735B",
"1983ApJ...270..365M",
"1989MNRAS.241..849O",
"1990ApJ...356..359H",
"1991RMxAA..22..255A",
"1992MNRAS.254..466P",
"1994ApJ...427L...1F",
"1994Natur.370..629M",
"1996ApJ...462..563N",
"1996MNRAS.278..488Z",
"1998ARA&A..36..435M",
"1998ApJ...501L..33B",
"1999AJ....118.1671B",
"2000ApJ...531..727O",
"2000ApJ...536L..39D",
"2004AJ....127..832C",
"2004AJ....128..687D",
"2005AJ....129.1443C",
"2006AJ....131..912O",
"2006ApJ...652..306S",
"2006MNRAS.368.1073G",
"2006MNRAS.373.1451R",
"2007ApJ...663..948G",
"2008ApJ...678..780G",
"2009ApJ...699.1389C",
"2009ApJ...704.1274W",
"2009MNRAS.396..887A",
"2010MNRAS.406.1220W",
"2011ApJ...742...20W",
"2012A&A...544L..14L",
"2012ApJ...745...83A",
"2012ApJ...746...89J",
"2012ApJ...756L..18Y",
"2012MNRAS.421.3464P",
"2012MNRAS.426..601C",
"2013ApJ...763...62A",
"2013JCoPh.236..580C",
"2014ApJ...782L..39A",
"2014ApJ...785...51A",
"2014MNRAS.444.3738A",
"2015ApJ...806..220A",
"2015MNRAS.454.3778P"
] | [
"10.1093/mnras/stw1647",
"10.48550/arXiv.1607.01526"
] | 1607 | 1607.01526_arXiv.txt | The Fornax galaxy is the brightest dwarf spheroidal galaxy (dSph) satellite of the Milky Way (MW) and is, among all of the MW satellites, the only one that hosts five globular clusters, located within $1$ kpc from its center, named Fornax 1,2,3,4 and 5. Moreover, Fornax does not show any bright nucleus. As the other MW satellites, Fornax is believed to reside within a dark matter halo, since its internal velocities are larger than expected from the measurement of the luminous mass \citep{mateo}. The presence of these five, metal poor and old, clusters in Fornax, with ages greater than 10 Gyr \citep{lrs}, represents a still open puzzle because the dynamical friction process (df) as estimated according to the overall galaxy characteristics should have already dragged all the clusters toward the galactic center. Actually, early calculations of the dynamical friction decay time for Fornax lead to few Gyr \citep{tremaine, ohlirich} . This is often referred to as the ``timing problem", which can be posed this way: if the clusters are in the final phase of their orbital decay we are in the unlikely state of looking at them just before their final sink to the galactic center. To study, and hopefully solve, the Fornax puzzle the minimal ingredients are (i) a reliable description of the dynamical friction process in this specific context and (ii) a detailed as possible definition of the phase space profile of the galaxy. With regard to the first point, we remind that the dynamical friction effect is often estimated by mean of the classical Chandrasekhar's formula in its local approximation \citep{Cha43I}, which is well suited to describe the dynamics of massive bodies traveling an extended, isotropic, system, only. It has been indeed proved that Chandrasekhar's approximation fails in more general cases, where more suited approximations have been proposed, as those by \cite{Bin77} and \cite{Pes92} for axisymmetric and triaxial systems, or by \cite{AntMer12} and \cite{ascd14df} for spherical, but cuspy, profiles. On another side, to have a meaningful description of the motion of its clusters, a detailed knowledge of the dynamical structure of Fornax is also required. At this regard, although this galaxy (like all the dSphs) is believed to have a massive dark matter halo (DMH), as expected on the base of the $\Lambda$-CDM paradigm, the density profile compatible with available kinematical data for Fornax does not seem to match the DMH profiles predicted from that paradigm \citep{walker}. Indeed, while CDM predicts the formation of haloes with density profiles scaling as $\rho(r)\propto r^{-1}$ \citep{NFW96}, the kinematic data available for the Fornax clusters suggest flatter density profiles \citep{flores,moore,gilmore,cowsik,jardel}. Many authors provided several explanations for the structure of Fornax and its GCs dynamics. As example, while some authors claim that the galaxy has a flat profile, with a core extended out to $300$ pc from the center \citep{strigari}, others propose that supernovae events could have injected sufficient energy to the environment to remove the DM cusp, leaving a cored profile \citep{pontzen12}. Moreover, due to the recent discovery of shell-like overdensities in that galaxy, some authors have proposed that Fornax is the result of a collision with a smaller galaxy \citep{olsew,coleman04,yozin}. On another side, \cite{angus}, argued that Modified Newtonian Dynamics (MOND, \citep{milgrom83}), can explain the observed dynamical features of the Fornax GCs. \cite{Goerdt} and \cite{Read} showed, via numerical simulations, that in cored profiles a massive body would experience an initial phase in which df is larger than that evaluated with the local approximation formula, going to a second phase in which the test particle stalls. Furthermore, \cite{cole} showed that the timing problem could be solved either by df stall in a cored profile or by the hypothesis that the clusters have formed out of Fornax. The first possibility stands upon the discovery that df becomes unefficient when the infalling object moves on an orbit which encloses a mass nearly equal to the object mass \citep{Gual08,ascd14df}. In this case, the objects reach a nearly stable orbit, never approaching the innermost region of the host galaxy. The second possibility, instead, requires that the GCs have formed in a peripheral region of the MW, and that they have been later tidally captured by Fornax. We stress that the study of the Fornax GC system would help to unveil the mistery hidden in the dynamics of dwarf galaxies, since a convincing explanation of the timing problem would lead to an improvement in the knowledge of the structure of dwarf galaxies, including its dark matter content. In this paper we investigate by mean of high resolution, direct, $N$-body simulations, the effect of the combined tidal field of the host Fornax galaxy and of the MW on the dynamics of the Fornax clusters. By mean of realistic estimates of the df decay times, we could provide a set of possible initial conditions to use in the direct $N$-body simulations. Later on, using reliable models for the Fornax dSph and considering either the case of a cored and a cuspy density profile, we simulated the dynamics of the Fornax GCs, along the motion of Fornax around the Milky Way host. The paper is organized as follows: in Section \ref{model} we present the models for the Galactic tidal field as well as the known properties of the Fornax orbit and the model for the Fornax galaxy and its globular cluster system. Section \ref{df} introduces semi-analytical estimates for the dynamical friction timescales, to place constraints on the cluster initial conditions to use in $N$-body simulations. Section \ref{nb} is devoted to present and discuss results of our direct $N$-body simulations; finally, in Section \ref{end} we draw the conclusions. | \label{end} In this paper we revisited the so called ``timing problem'' for the stellar clusters in the Fornax dwarf spheroidal galaxy. Using two complementary approaches, a simple semi-analytic investigation of the dynamical friction decay times and a more sophisticated and detailed series of numerical simulations for the clusters motion in Fornax as a satellite of the Milky Way, we obtained results which can be summarized as follows: \begin{itemize} \item we found that the missing orbital decay of the Fornax GCs is compatible either with a shallow profile or a steep cusp in the Fornax density profile. This means that a standard CDM density profile cannot be excluded; \item in the extreme hypothesis that the present 3D positions of the GCs coincide with their projected positions, we found that all the clusters should have formed within the Fornax tidal radius, quite independently of the Fornax density profile, even in the case of the most massive cluster (GC3); \item we investigated the gravitational effects induced by the MW tidal field using a series of detailed $N$-body simulations focused on nearly circular orbits. Our results show that in the majority of the investigated cases ($60\%$), the MW tidal field shorten the decay time-scale, leading to its decrease of a factor up to $15\%$; \item on the other hand, we have also found a significant fraction of cases ($35\%$) in which the MW acts against dynamical friction, increasing the decay time, and a small fraction of cases ($5\%$) where the GC is tidally captured by the MW; \item the previous points highlight the importance of both the MW tidal field and of the GCs ICs. Indeed, if the clusters were born in an outer region of Fornax, the MW tidal field tends to slow down their orbital decay but, on the other hand, the MW makes the orbital decay faster for GCs initially moving on orbits within the Fornax scale-radius; \item the MW tidal field induces the formation of tidal tails around Fornax, containing clumps whose surface densities are about 10 times higher than the density of the surrounding tail; \item if the GCs move on nearly circular orbits, there is a wide range of ICs for which they can survive up to a Hubble time, even in the case of a steep Fornax density distribution, thus providing a satisfactory solution to the timing problem and making extremely hard to discern about the shape of possible different mass distributions for Fornax. \end{itemize} In conclusion, this paper shows that the timing problem for the Fornax GCs can be solved even in the case that Fornax has a steep density profile, unless the GCs started moving, at their birth, on nearly radial orbits. Moreover, we have demonstrated that the Galactic gravitational field affects marginally the results, leading in general to shorter decay times in dependence on the IC set. On the other hand, we notice that in few cases, some GCs have been tidally captured by the MW. As a side effect, our results indicate that a standard dark matter mass distribution cannot be completely excluded for Fornax on the base of its GC dynamics. | 16 | 7 | 1607.01526 | The Fornax dwarf spheroidal galaxy is the most massive satellites of the Milky Way, claimed to be embedded in a huge dark matter halo, and the only among the Milky Way satellites hosting five globular clusters. Interestingly, their estimated masses, ages and positions seem hardly compatible with the presence of a significant dark matter component, as expected in the ΛCDM scheme. Indeed, if Fornax would have a CDM halo with a standard density profile, all its globular clusters should have sunk to the galactic centre many Gyr ago due to dynamical friction. Due to this, some authors proposed that the most massive clusters may have formed out of Fornax and later tidally captured. In this paper, we investigate the past evolution of the Fornax GC system by using both a recently developed, semi-analytical treatment of dynamical friction and direct N-body simulations of the orbital evolution of the globular clusters within Fornax and of Fornax galaxy around the Milky Way. Our results suggest that an `in situ' origin for all the clusters is likely if their observed positions are close to their spatial ones and their orbits are almost circular. Moreover, the Milky Way seems to accelerate the GC decay reducing the decay time of 15 per cent. Nevertheless, our results indicate that the GCs survival probability exceeds 50 per cent, even in the case of cuspy density profiles. We conclude that more detailed data are required to shed light on the Fornax dark matter content, to distinguish between a cuspy or a cored profile. | false | [
"cuspy density profiles",
"dynamical friction",
"Fornax GC",
"Fornax",
"the Fornax dark matter content",
"five globular clusters",
"the globular clusters",
"direct N-body simulations",
"the Fornax GC system",
"a huge dark matter halo",
"a standard density profile",
"the Milky Way satellites",
"a significant dark matter component",
"GC",
"positions",
"all its globular clusters",
"a cored profile",
"the Milky Way",
"the orbital evolution",
"spheroidal galaxy"
] | 10.009286 | 7.42452 | 167 |
5209849 | [
"Colombo, S.",
"Orlando, S.",
"Peres, G.",
"Argiroffi, C.",
"Reale, F."
] | 2016A&A...594A..93C | [
"Impacts of fragmented accretion streams onto classical T Tauri stars: UV and X-ray emission lines"
] | 15 | [
"INAF-Osservatorio Astronomico di Palermo, Piazza del Parlamento 1, 90134, Palermo, Italy",
"INAF-Osservatorio Astronomico di Palermo, Piazza del Parlamento 1, 90134, Palermo, Italy",
"INAF-Osservatorio Astronomico di Palermo, Piazza del Parlamento 1, 90134, Palermo, Italy; Dipartimento di Fisica & Chimica, Università degli Studi di Palermo, Piazza del Parlamento 1, 90143, Palermo, Italy",
"INAF-Osservatorio Astronomico di Palermo, Piazza del Parlamento 1, 90134, Palermo, Italy; Dipartimento di Fisica & Chimica, Università degli Studi di Palermo, Piazza del Parlamento 1, 90143, Palermo, Italy",
"INAF-Osservatorio Astronomico di Palermo, Piazza del Parlamento 1, 90134, Palermo, Italy; Dipartimento di Fisica & Chimica, Università degli Studi di Palermo, Piazza del Parlamento 1, 90143, Palermo, Italy"
] | [
"2017A&A...598L...8P",
"2017A&A...602A..10G",
"2017A&A...607A..14A",
"2017SciA....3E0982R",
"2018MNRAS.475.4367D",
"2019A&A...624A..50C",
"2019A&A...629L...9C",
"2019A&A...630A..84D",
"2019ASPC..519...21I",
"2019ASSP...55...29D",
"2019ASSP...55...43O",
"2019arXiv190409156D",
"2020A&A...640A.128R",
"2020Galax...8...27S",
"2022hxga.book...57S"
] | [
"astronomy"
] | 3 | [
"accretion",
"accretion disks",
"magnetohydrodynamics (MHD)",
"stars: pre-main sequence",
"shock waves",
"X-rays: stars",
"ultraviolet: stars",
"Astrophysics - Solar and Stellar Astrophysics"
] | [
"1962pfig.book.....S",
"1977ApJ...211..135C",
"1984ApJ...277..605G",
"1988ApJ...330..350B",
"1991ApJ...370L..39K",
"1993ApJ...404..625D",
"1996JGR...10124443O",
"1999JCoPh.149..270B",
"2002ApJ...567..434K",
"2007A&A...465L...5A",
"2007ApJS..170..228M",
"2008A&A...491L..17S",
"2008MNRAS.388..357K",
"2010A&A...510A..71O",
"2010A&A...522A..55S",
"2010ApJ...710.1835B",
"2011A&A...526A.104C",
"2012ApJ...752..100A",
"2013A&A...557A..69M",
"2013A&A...559A.127O",
"2013ApJ...763...86L",
"2013ApJS..207....1A",
"2013Sci...341..251R",
"2014ApJ...795L..34B",
"2014ApJ...797L...5R",
"2017A&A...597A...1C"
] | [
"10.1051/0004-6361/201628858",
"10.48550/arXiv.1607.03009"
] | 1607 | 1607.03009_arXiv.txt | Classical T Tauri Stars (CTTS) are young stars surrounded by \salvo{an accretion} disk. \salvo{According to the magnetospheric accretion scenario, gas from the disk accretes onto the star through accretion columns \citep{1991Apj...370L..39K}. Several lines of evidence support this picture especially in the optical and infrared bands (e.g. \citealt{1988ApJ...330..350B}).} Also accreting T Tauri Stars show a \salvo{soft X-ray} (0.2-0.8 KeV) excess, with typical lines produced at \salvo{temperatures} $10^5-10^6$ K. \salvo{This excess has been interpreted as due to impacts of the accreting material with the stellar surface where a shock is produced and dissipates the kinetic energy of the downfalling material \citep{1991Apj...370L..39K}. The shock heats the plasma up to temperatures of few million degrees, causing X-ray emission \citep{2002ApJ...567..434K, 2007A&A...465L...5A}. The heated plasma is characterized by density of $n\approx 10^{11}-10^{13}$ cm$^{-3}$ (e.g. \citealt{2007A&A...465L...5A}).} \salvo{The interpretation of the soft X-ray excess in CTTSs in terms of accretion shocks is well supported by hydrodynamic (HD) and magnetohydrodynamic (MHD) models.} {Time-dependent 1D model of radiative accretion shocks in CTTSs provided a first accurate description of the dynamics of the postshock plasma \citep{2008MNRAS.388..357K,2008A&A...491L..17S}. In particular \cite{2008A&A...491L..17S} proposed a one.dimensional hydrodynamic (HD) model of a continuous accretion flow impacting onto the chromosphere of a CTTS, thus assuming the ratio between the thermal pressure and the magnetic pressure $\beta <<1$. Their model reproduced the main features of high spectral resolution X-ray observations of the CTTS MP Mus that was previously interpreted as due to postshock plasma \citep{2007A&A...465L...5A} } \salvo{More recently 2D MHD models of accretion impacts have been studied (\citealt{2010A&A...510A..71O, 2013A&A...557A..69M, 2013A&A...559A.127O}) to explore those cases where the low-$\beta$ approximation cannot be applied (and, therefore, the 1D models cannot be used). These models have shown that the accretion dynamics can be complex with the structure and stability of the impact region of the stream strongly depending on the configuration and strength of the stellar magnetic field. Depending on the magnetic field strength, the atmosphere around the impact region can be also perturbed, leading to accreting plasma leaks at the border of the main stream.} \salvo{Although HD and MHD models of accretion shocks have provided a theoretical support to the hypothesis that the soft X-ray excess in CTTSs originates from impacts of accretion columns onto the stellar surface, several points still remain unclear. Some of these points concern the emission in the UV band arising from impact regions. There is} evidence that \salvo{a significant amount of plasma at $10^{5}$ K (much larger than expected from current models; Costa et al. 2016, submitted)} \salvo{is} produced \salvo{in the accretion process}. \cite{2013ApJS..207....1A} analyzed UV spectra collected with the {\it Hubble Space Telescope} (HST) of 28 T Tauri stars and studied the C\,IV doublet at 1550 \AA. They found that each component of the doublet is described by 2 Gaussian components with different speed and width. \salvo{About half of their sample exhibits line profiles analogous (but with different Doppler shifts) to those of TW Hya that consist of a narrow component redshifted at speeds of $\approx 30$ km s$^{-1}$ (positive speed indicates material that falls into the star) and a broader component centered at $\approx 120$ km s$^{-1}$ and with the redshifted wing reaching $\approx 400$ km s$^{-1}$. The latter component cannot be explained, as post-shock emission, with current models of a continuous accretion stream: assuming a free fall velocity of $\approx 500-600$ km s$^{-1}$ and a strong shock, the velocity of the post-shock plasma cannot be larger than $\approx 100-120$ km s$^{-1}$ \citep{2010A&A...522A..55S}.} \salvo{Recently \cite{2014ApJ...797L...5R} proposed an explanation on the origin of the observed asymmetries and redshifts of UV emission lines in CTTSs. These authors studied the impacts of dense plasma fragments falling back on the surface of the Sun after a violent eruption occurred on 2011 June 7, showing that this phenomenon reproduces on the small scale accretion impacts onto CTTSs (see also \citealt{2013Sci...341..251R}). They modeled the impacts with HD simulations and synthesized the emission in UV and X-ray bands. They found that UV emission may originate from the shocked front shell of the still downfalling fragments, thus producing a broad redshifted component in UV lines up to speeds around $\approx 400$ km s$^{-1}$.} \salvo{In this work we investigate further the scenario of a fragmented stream through MHD modeling. More specifically we study the structure and stability of the post-shock plasma after the impact of a clumpy or fragmented stream onto the stellar surface. We investigate the origin of UV and X-ray emission at impact regions and if and how the stream fragmentation can be responsible of the observed asymmetries and redshifts of UV emission lines in CTTSs. To this end, we developed an MHD model describing an accretion column consisting of several high density blobs which impact onto the chromosphere of a CTTS. We synthesized the C\,IV (1550 \AA) and O\,VIII (18.97 \AA) emission lines, including the effect of Doppler shift due to plasma motion along the line of sight. The paper is structured as follow: in Sect.~\ref{sec2} we describe the model and the synthesis of emission lines; in Sect.~\ref{sec3} we discuss the results of the simulations and the synthesis of emission lines; and finally in Sect.~\ref{sec4} we drawn our conclusions.} | \label{sec4} In this work we investigated the effects of \salvo{stream fragmentation} on C\,IV and O\,VIII emission lines. We developed a model that describes \salvo{a stream composed by a series of dense blobs} impacting onto the surface of a CTTS. Our model \salvo{takes} into account the stellar magnetic field, the gravity, the radiative \salvo{losses} from optically thin plasma and the thermal conduction. \salvo{The aim was to explore if and how the impact of a fragmented stream onto the chromosphere of a CTTS reproduces profiles of C\,IV doublet similar to those observed by \cite{2013ApJS..207....1A}. Our main findings can be summarized as follow:} \begin{itemize} \item \salvo{The impact of a series of dense blobs onto the stellar surface produces a more complex post-shock region than that in the case of a continuous stream. In particular the blob impacts produce strong shocks propagating through the blobs and then upflows after the blobs are fully shocked. The upflows in turn hit and shock the still downfalling blobs, producing a large variety of plasma structures (knots, filaments) differing in density, temperature, and downfalling velocity. These structures are not present in the case of a continuous stream.} \item \salvo{If the stream is fragmented the C\,IV (1550 \AA) lines have a highly asymmetric and broad profiles. The lines split into a narrow and intense component redshifted to speed $\approx 50$~km~s$^{-1}$ and a multitude of faster components redshifted to speeds in the range between 200 and 400~km~s$^{-1}$. The narrow component originates from the post-shock plasma at the base of the accretion column which cools down under the effect of thermal conduction and radiative losses. The fast components originate from thermal instabilities occurring at high altitudes in the shocked stream and from the plasma structures forming during the interaction of upflowing surges and downfalling blobs. This is in agreement with \cite{2014ApJ...797L...5R}.} \item \salvo{The intensity and velocity of the fast components depend on the stream fragmentation: the finer is the fragmentation, the more intense are the fast components. Assuming a more realistic scenario in which few accretion streams with different viewing angles (and therefore different line shifts) are present, the fast components easily merge together to form a single broad component redshifted to speed $\approx 250$~km~s$^{-1}$. A similar result has been found by \cite{2014ApJ...797L...5R} by adopting an HD model to study blob falls on the solar surface. The narrow component at $\approx 50$~km~s$^{-1}$ and the broad component at $\approx 250$~km~s$^{-1}$ are analogous to those found by \cite{2013ApJS..207....1A} for most of the CTTSs of their sample. Thus we interpret the shape of observed C\,IV lines as evidence of density structured or fragmented accretion streams.} \item \salvo{The O\,VIII (18.97 \AA) lines have a symmetric observable profile redshifted to speed ranging between 100 and 200~km~s$^{-1}$. The redshift increases roughly with the level of stream fragmentation: the shift is the smallest in the case of a continuous flow and the largest for a train of small blobs. In any case our model predicts that accretion impacts would produce detectable shifts in the O\,VIII lines.} \item \salvo{As in the case of continuous accretion streams, models of fragmented streams reproduce quite well the luminosity of O\,VIII lines measured in CTTSs \citep{2007A&A...465L...5A,2010ApJ...710.1835B,2012ApJ...752..100A} and, in general, underestimate even by orders of magnitude the luminosity of C\,IV lines. On the other hand, we found that assuming an high level of stream fragmentation is in better agreement with observations. In these models, in fact, many interactions between upflowing surges and downfalling blobs are present which produce a multitude of plasma structures with different density and temperature. Many of them cool down because of radiative losses thus contributing to emission in C\,IV lines. We conclude that the stream fragmentation enhances the emission in the UV band.} \end{itemize} In conclusion, our models reproduce profiles of C IV and O VIII lines remarkably similar to those observed (\citealt{2013ApJS..207....1A}, Argiroffi in preparation) and suggest that the UV emission originates mainly from plasma structures developed as a result of the impact of a density structured or fragmented accretion stream. On the other hand our models predict in general UV luminosities lower than observed. We note that oru models assume that the plasma is optically thin in the whole domain. However, optically thick plasma, as that of the chromosphere and of the unshocked stream, is present around the impact region. This plasma, on one hand, absorb part of the X-ray emission arising from the post-shock plasma \citep{2010A&A...522A..55S,2014ApJ...795L..34B} and, on the other hand, can be heated up to $ \log T (K) \approx 5$ by irradiation by the post-shock plasma, thus contributing to UV emission (Costa et al. 2016, submitted). In this work we did not take into account the absorption by optically thick material and the effect of radiative heating of the unshocked stream by post-shock plasma. In a future work we plan to include these effects on the model to investigate more deeply the origin of UV emission arising from impact regions of fragmented accretion streams. | 16 | 7 | 1607.03009 | Context. The accretion process in classical T Tauri stars (CTTSs) can be studied through the analysis of some UV and X-ray emission lines which trace hot gas flows and act as diagnostics of the post-shock downfalling plasma. In the UV-band, where higher spectral resolution is available, these lines are characterized by rather complex profiles whose origin is still not clear. <BR /> Aims: We investigate the origin of UV and X-ray emission at impact regions of density structured (fragmented) accretion streams. We study if and how the stream fragmentation and the resulting structure of the post-shock region determine the observed profiles of UV and X-ray emission lines. <BR /> Methods: We modeled the impact of an accretion stream consisting of a series of dense blobs onto the chromosphere of a CTTS through two-dimensional (2D) magnetohydrodynamic (MHD) simulations. We explored different levels of stream fragmentation and accretion rates. From the model results, we synthesize C IV (1550 Å) and O VIII (18.97 Å) line profiles. <BR /> Results: The impacts of accreting blobs onto the stellar chromosphere produce reverse shocks propagating through the blobs and shocked upflows. These upflows, in turn, hit and shock the subsequent downfalling fragments. As a result, several plasma components differing for the downfalling velocity, density, and temperature are present altoghether. The profiles of C IV doublet are characterized by two main components: one narrow and redshifted to speed ≈ 50 km s<SUP>-1</SUP> and the other broader and consisting of subcomponents with redshift to speed in the range 200-400 km s<SUP>-1</SUP>. The profiles of O VIII lines appear more symmetric than C IV and are redshifted to speed ≈ 150 km s<SUP>-1</SUP>. <BR /> Conclusions: Our model predicts profiles of C IV line remarkably similar to those observed and explains their origin in a natural way as due to stream fragmentation. <P />Movies are available at <A href="http://www.aanda.org/10.1051/0004-6361/201628858/olm">http://www.aanda.org</A> | false | [
"line profiles",
"C IV line",
"stream fragmentation",
"O VIII lines",
"accretion rates",
"UV and X-ray emission lines",
"dense blobs",
"the post-shock downfalling plasma",
"C IV doublet",
"C IV",
"fragmentation",
"speed",
"shocked upflows",
"impact regions",
"several plasma components",
"profiles",
"accreting blobs",
"UV and X-ray emission",
"hot gas flows",
"the post-shock region"
] | 9.324092 | 12.202207 | 147 |
12586989 | [
"Schmidtmann, Birte",
"Winters, Andrew R."
] | 2017JCoPh.330..566S | [
"Hybrid entropy stable HLL-type Riemann solvers for hyperbolic conservation laws"
] | 7 | [
"MathCCES, RWTH Aachen University, Schinkelstr. 2, 52062 Aachen, Germany",
"Mathematisches Institut, Universität zu Köln, Weyertal 86-90, 50931 Köln, Germany"
] | [
"2019JCoPh.378..477S",
"2019ShWav..29..755P",
"2020JCoPh.41609545T",
"2021ApJS..257...24L",
"2021ApJS..257...34F",
"2022JCoPh.46811521W",
"2023JCoPh.49312505H"
] | [
"astronomy",
"physics",
"general"
] | 1 | [
"Entropy stability",
"Ideal magnetohydrodynamics",
"HLL",
"Riemann solver",
"Discrete entropy inequality",
"Mathematics - Numerical Analysis",
"Astrophysics - Instrumentation and Methods for Astrophysics"
] | [
"1999CRASM.328..479D",
"2003AcNum..12..451T",
"2003JPlPh..69..253T",
"2016JCoPh.304...72W",
"2017SJSC...39A2911S"
] | [
"10.1016/j.jcp.2016.10.034",
"10.48550/arXiv.1607.06240"
] | 1607 | 1607.06240_arXiv.txt | We consider the numerical solution of systems of hyperbolic conservation laws of the form \begin{equation} \label{eq:hypLaw} \pderivative{\vec{q}}{t} + \nabla\cdot\vec{f} = 0, \end{equation} on a domain $\Omega$. For a one-dimensional approximation we divide $\Omega$ into $K$ non-overlapping grid cells $C_i = [x_{i-1/2},\; x_{i+1/2}],\, i= 1,\ldots, K$ which are not necessarily equidistant. In the context of finite volume schemes, hyperbolic equations, such as \eqref{eq:hypLaw}, require a numerical flux function which fully determines the properties of the scheme \cite{LeVeque2002}. The numerical flux function takes as input the left and right value of $\vec{q}$ at the cell interface and solves a local Riemann problem. Smooth initial flows governed by \eqref{eq:hypLaw} may develop discontinuities (\eg shocks) in finite time. Thus, solutions are sought in the weak sense \cite{LeVeque2002}. However, weak solutions are not unique and need to be supplemented with additional admissibility criteria. Following the work of \eg \cite{Winters2016,Tadmor2003}, we use the concept of entropy to construct discretizations that agree with the second law of thermodynamics. That is, the numerical flux function will possess entropy stability, cf. \cite{Tadmor2003} and references therein. In particular, we prove entropy stability for the HLL scheme and present the construction of HLL-type entropy stable numerical flux functions. It is known that HLL-type schemes are more dissipative than upwind schemes. However, HLL-type methods need less information about the eigendecomposition of the flux Jacobian. This is advantageous because the eigenstructure might be computationally expensive or no analytical expression exists, especially for large systems. As such, we consider three standard dissipation terms, namely Lax-Friedrichs (LF), HLL, and Lax-Wendroff (LW) and present two hybrid dissipation terms introduced in \cite{Schmidtmann2016}. We demonstrate that these five schemes are provably entropy stable. % The paper is organized as follows: Sec.~\ref{scn:ESSolvers} provides a brief background on entropy stable numerical fluxes. In Sec.~\ref{scn:Riemann} we show entropy stability for the LF, HLL, and LW dissipation terms. The creation of two new hybrid entropy stable dissipation operators is shown in Sec.~\ref{scn:HybridRiemann}. We demonstrate in Sec.~\ref{sec:NumSim} that the new hybrid numerical flux reduce the overall dissipation in a standard finite volume scheme. Our conclusions and outlook are drawn in the final section. | \label{scn:Conclusion} In this work we constructed two one-parameter families of hybrid entropy stable numerical fluxes. An advantage of the new numerical flux functions is that they remain applicable even when the eigenstructure of the flux Jacobian matrix is unknown. % The derivations and proofs in this work are kept general such that the hybrid entropy stable solvers can be applied to a broad range of non-linear hyperbolic conservation laws. As an example, we applied the novel numerical fluxes to the ideal MHD equations and demonstrated the decreased magnitude of dissipation for the hybrid solvers versus a standard solver. % In the future we plan to apply the hybrid entropy stable Riemann solvers to other complex hyperbolic systems, such as the two-layer shallow water or the regularized 13-Moment Equations of Grad. | 16 | 7 | 1607.06240 | It is known that HLL-type schemes are more dissipative than schemes based on characteristic decompositions. However, HLL-type methods offer greater flexibility to large systems of hyperbolic conservation laws because the eigenstructure of the flux Jacobian is not needed. We demonstrate in the present work that several HLL-type Riemann solvers are provably entropy stable. Further, we provide convex combinations of standard dissipation terms to create hybrid HLL-type methods that have less dissipation while retaining entropy stability. The decrease in dissipation is demonstrated for the ideal MHD equations with a numerical example. | false | [
"hyperbolic conservation laws",
"entropy stability",
"characteristic decompositions",
"standard dissipation terms",
"less dissipation",
"large systems",
"Jacobian",
"HLL",
"greater flexibility",
"dissipation",
"several HLL-type Riemann solvers",
"hybrid HLL-type methods",
"HLL-type schemes",
"HLL-type methods",
"schemes",
"convex combinations",
"Riemann",
"MHD",
"a numerical example",
"the ideal MHD equations"
] | 8.789782 | 4.468701 | 28 |
168897 | [
"Sayanagi, Kunio M.",
"Dyudina, Ulyana A.",
"Ewald, Shawn P.",
"Fischer, Georg",
"Ingersoll, Andrew P.",
"Kurth, William S.",
"Muro, Gabriel D.",
"Porco, Carolyn C.",
"West, Robert A."
] | 2013Icar..223..460S | [
"Dynamics of Saturn’s great storm of 2010-2011 from Cassini ISS and RPWS"
] | 74 | [
"Department of Atmospheric and Planetary Sciences, Hampton University, Hampton, VA 23668, USA; Division of Geological and Planetary Sciences, California Institute of Technology, Pasadena, CA 91125, USA",
"Division of Geological and Planetary Sciences, California Institute of Technology, Pasadena, CA 91125, USA",
"Division of Geological and Planetary Sciences, California Institute of Technology, Pasadena, CA 91125, USA",
"Space Research Institute, Austrian Academy of Sciences, A-8042 Graz, Austria; Department of Physics and Astronomy, University of Iowa, Iowa City, IA 52242, USA",
"Division of Geological and Planetary Sciences, California Institute of Technology, Pasadena, CA 91125, USA",
"Department of Physics and Astronomy, University of Iowa, Iowa City, IA 52242, USA",
"Division of Geological and Planetary Sciences, California Institute of Technology, Pasadena, CA 91125, USA; Department of Earth and Planetary Sciences, University of California Santa Cruz, Santa Cruz, CA 95064, USA",
"Cassini Imaging Central Laboratory for Operations, Space Science Institute, Boulder, CO 80301, USA",
"Jet Propulsion Laboratory, California Institute of Technology, Pasadena, CA 91109, USA"
] | [
"2013Icar..226..641L",
"2013Icar..226.1020D",
"2013JGRA..118.7246C",
"2013PhFl...25f6604S",
"2014AnGeo..32.1463F",
"2014ApJ...786...92A",
"2014Icar..229..170S",
"2014Icar..238..110G",
"2014Icar..241..313D",
"2014Icar..242..122T",
"2014MNRAS.444.1369H",
"2014Natur.505..625S",
"2015A&A...580A..55C",
"2015GeoRL..42.2144L",
"2015Icar..252..121D",
"2015Icar..254...72F",
"2015Icar..261..149M",
"2015JGRE..120..155A",
"2015LPI....46.2606W",
"2015P&SS..113..100P",
"2015PASP..127..941C",
"2016Icar..264..137F",
"2016Icar..271..222D",
"2016Icar..271..400B",
"2016Icar..276..141S",
"2016Icar..277....1P",
"2016Icar..277..196F",
"2016Icar..278..215O",
"2016JGRE..121.1814T",
"2016MmSAI..87...46M",
"2016NatCo...713262S",
"2016PASP..128a8005N",
"2016PASP..128a8008T",
"2016ewr..book.....S",
"2017A&G....58d4.20C",
"2017Icar..285...68S",
"2017Icar..286...94F",
"2017Icar..286..241G",
"2017pre8.conf..223M",
"2018ASPC..513..131S",
"2018ConPh..59..251K",
"2018GeoRL..45.6823S",
"2018GeoRL..45.7399G",
"2018Icar..302..360S",
"2018Icar..302..499D",
"2018arXiv180408340G",
"2019A&A...621A.113F",
"2019ApJ...881..142W",
"2019JFM...859..204D",
"2019JGRE..124.3041L",
"2019NCimR..42..197F",
"2019Sci...364.1046S",
"2020ApJS..247...58W",
"2020Icar..33513377S",
"2020Icar..33613429H",
"2020NatAs...4..180S",
"2020SSRv..216..122I",
"2021ApJ...923..138C",
"2021GeoRL..4893982W",
"2021Icar..35414095B",
"2021Icar..36914650L",
"2021JFM...909A...4H",
"2021PSJ.....2...47S",
"2021arXiv210914289C",
"2022ExFl...63...76L",
"2022Icar..37914942H",
"2022RemS...15..219P",
"2023A&ARv..31....5S",
"2023Icar..38915228G",
"2023JGRE..12807924F",
"2023RemS...15..702W",
"2023SciA....9G9419L",
"2024NatCo..15.5045W",
"2024SSRv..220...15W"
] | [
"astronomy",
"earth science"
] | 18 | [
"Astrophysics - Earth and Planetary Astrophysics"
] | [
"1949JAtS....6..105K",
"1973Icar...20..465W",
"1982Natur.297..132H",
"1982Sci...215..504S",
"1983JGR....88.8650S",
"1986Icar...65..335L",
"1988JAtS...45.1380D",
"1989NASSP.494..245B",
"1989Sci...245.1367H",
"1991Natur.353..397S",
"1991Sci...254..684S",
"1992Icar...95..163B",
"1992Icar..100..485W",
"1993Icar..105..110S",
"1993JGR....9818857S",
"1994Chaos...4..341S",
"1995AnRFM..27..293D",
"1996Icar..121....1S",
"1996Sci...271..631S",
"1996Sci...272..854R",
"1998Icar..135..230B",
"1999Icar..137...24A",
"1999P&SS...47.1277S",
"2000Icar..147..405S",
"2001Icar..152..316G",
"2001Icar..154..459S",
"2004SSRv..115..363P",
"2005Sci...307.1243P",
"2006DPS....38.1121M",
"2006GeoRL..33.3201S",
"2006Icar..180..161P",
"2006Icar..183..135F",
"2006Icar..185..430S",
"2006Icar..185..558S",
"2006JGRE..111.5004V",
"2007CeMDA..98..155S",
"2007Icar..187..520S",
"2007Icar..188...35C",
"2007Icar..189..479D",
"2007Icar..190..545D",
"2007Icar..191..665G",
"2008AJ....135.2446C",
"2008SSRv..137..257Z",
"2009Icar..203..164A",
"2009Icar..203..499H",
"2009P&SS...57.1650B",
"2009P&SS...57.1682R",
"2010Icar..205..674K",
"2010Icar..207..359C",
"2010Icar..209..665D",
"2010Icar..210..202S",
"2010P&SS...58.1475W",
"2011Icar..215...62G",
"2011Natur.475...71S",
"2011Natur.475...75F",
"2011Sci...332.1413F",
"2012ApJ...760...24H",
"2012Icar..220..561S",
"2012Icar..221..560F"
] | [
"10.1016/j.icarus.2012.12.013",
"10.48550/arXiv.1607.07246"
] | 1607 | 1607.07246_arXiv.txt | } Cumulus convecting storms on Saturn are known for their episodic behavior. Convective events on Saturn can be identified visually and through radio noise. The events occur intermittently within a small range of latitudes and are interspersed with quiescent periods that last for a year or longer \citep{Sromovsky_etal_1983, Dyudina_etal_2007, DelGenio_etal_2007}. The largest of these encircle the planet and are sometimes called Great White Spots \citep{Sanchez-Lavega_1994}. These giant storms exhibit a $\sim$30-year periodicity ($\sim$1~Saturn Year), and have been recorded in 1876, 1903, 1933, 1960 and 1990, in which the storms lasted for 26, 150, 44, 41, and 55 days, respectively \citep{Alexander_1962, Sanchez-Lavega_1994}. All documented planet-encircling storms have occurred in the northern hemisphere, alternately erupting in mid-latitude and equatorial regions \citep{Alexander_1962, Sanchez-Lavega_1994}. These storms exhibit some morphological similarities to Jupiter's South Equatorial Belt (SEB) revival events \citep{Sanchez-Lavega_Gomez_1996}; however, their convective areas occupy an area much narrower than the surrounding jets, and move at speeds that reflect the background zonal mean wind. An SEB revival event may last up to 7 months; however, unlike Saturn's planet-encircling storms, its relatively small convective cores do not form a large organized structure. The 1990 equatorial planet-encircling storm of Saturn was an early scientific target of the Hubble Space Telescope \citep{Sanchez-Lavega_etal_1991, Sanchez-Lavega_1994, Beebe_etal_1992, Westphal_etal_1992}. The data collected during the 1990 storm enabled examinations of Saturn's equatorial dynamics. Based on comparing the equatorial wind speeds between Voyager and Cassini measurements, \cite{Perez-Hoyos_Sanchez-Lavega_2006} suggested that the 1990 storm caused a deceleration in the equatorial jet. Numerical simulations by \cite{Sayanagi_Showman_2007} tested the hypothesis that the storm caused a deceleration. They demonstrated that the observed apparent wind change can be explained by the deceleration of the wind caused by the storm in combination with the change in the altitudes of the tracked clouds. This interpretation is consistent with the radiative transfer analysis of \cite{Perez-Hoyos_Sanchez-Lavega_2006}. A new planet-encircling storm started on December~5, 2010. The 2010-2011 outburst was the first planet-encircling storm to be studied from a spacecraft in orbit around Saturn. The Cassini orbiter's Radio and Plasma Wave Science (RPWS) instrument detected radio pulses emitted by lightning discharges in the storm \citep{Fischer_etal_2011Nature}, and the Composite Infrared Spectrometer (CIRS) measured the storm's large-scale heating of the stratosphere and a longitudinal thermal perturbation that is consistent with the formation of a new anticyclonic vortex \citep{Fletcher_etal_2011Science, Fletcher_etal_2012}. Also, ground-based observations captured the development of the large scale disturbance \citep{Sanchez-Lavega_etal_2011Nature}. The mechanism that controls the intermittent appearances and scales of these extreme outbursts is unknown; a better understanding of the conditions that precede these episodes is one step toward unveiling the storms' origin. Here, we analyze Cassini ISS images and RPWS data to investigate the temporal evolution of the 2010-2011 planet-encircling storm. Our observations cover the temporal evolution of the planetary-scale convective disturbance from its beginning on December~5, 2010 until the end of 2011. The rest of our report is organized as follows. Section~2 describes the ISS image sets and the processing applied to them in our study. Section~3 presents the chronological development of the 2010-2011 storm. Section~4 summarizes our findings. | We have reported the evolution of Saturn's latest planet-encircling storm of 2010-2011 using two of the instruments aboard Cassini spacecraft, ISS and RPWS. Our observational coverage enabled us to study the preconditions of the storm, in which we show that the storm erupted out of a previously known feature called the String of Pearls (SoPs). After the storm erupted on December~5, 2010, the outburst grew and engulfed the entire latitude zone. By January 11, 2011, the storm developed a well defined structure with three primary parts as shown in Fig.~5. The western most feature is the particularly bright \emph{head} that propagated at an average rate of $-$2.79$\pm$0.08 \degree~day$^{-1}$ ($-$26.9$\pm$0.8~m~s$^{-1}$), and its longitudinal position is well described by Eq.~\ref{e:head_motion}. A bright body of cloud followed to the east of the head, which is bounded to the east by a new large anticyclonic vortex (AV) that was spawned by the storm. To the east of the AV, a turbulent pattern of clouds formed. The AV maintained a mean drift speed of $-$0.85\degree~day$^{-1}$ ($-$8.4~m$^{~}$s$^{-1}$) between December~24, 2010 and June~14, 2011; during this period, the center of AV did not deviate from Eq.~\ref{e:AV_motion} by more than 10\degree~in longitude (Fig.~17a). We performed cloud-tracking wind measurement on the AV, and showed that its tangential wind speed reached 100~m~s$^{-1}$; we also measured that the relative vorticity within the vortex to be $-$6$\pm$1$\times$10$^{-5}$~s$^{-1}$. With the east-west diameter of 12,000~km, the new anticyclonic vortex became greater in size than any tropospheric vortex previously seen on Saturn. The rapid growth and shrinkage of the new anticyclone is in a stark contrast to the steadiness of anticyclonic vortices on Jupiter. Oval BA today is a result of many dynamical changes over the last $\sim$70 years; however, it has mostly been growing in size through mergers, and the precursor vortices also stayed steady for over 50~years until the mergers. Compared to Jupiter, Saturn has been known to harbor far fewer vortices \citep{Vasavada_etal_2006}. Our analysis suggests that Saturn's inability to maintain even the largest of the vortices contributes to this difference between Jupiter and Saturn. Even though the new Saturnian anticyclone emerged in a highly disturbed region, we do not expect the turbulence to have contributed to its inability to stay large because Jupiter's GRS, for example, resides in the most turbulent latitude of Jupiter and yet it has persisted at least since 1879, and possibly nearly 400 years \citep{Hooke_1665, Beebe_Orton_West_1989}. Lastly, we note that a stratospheric vortex larger in size than the AV emerged in the aftermath of the storm, which persists as of late 2012 \citep{Fletcher_etal_2012DPS}; its future evolution will be of an interest in the context of Saturnian vortex dynamics. The storm underwent a major change in its dynamics after around June~20, 2011 when the storm's head collided with the anticyclone after the anticyclone's longitude trailed 360\degree~longitude behind the core. After the collision, the storm's convective activity displayed a major decline. The SED signals emitted by the storm's lightning activities followed the growth and demise of the cumulus storm observed in the ISS images until the head-AV collision in mid-June. The head-AV collision marked a sharp decline in the SED activities detected by the RPWS. In late August 2011, the SED signal resurged for 9~days, and we identified the source clouds in the ISS images. The RPWS detected two more weak SED activities for the remainder of 2011 during September 30 - October 6, and December 26-28. The storm left the region between 25\degree N and 40\degree N in a highly disturbed state. After the storm, a region that appears particularly dark in CB2 channel emerged between 31\degree N - 38\degree N latitude spanning up to 180\degree~in longitude. After the storm, this region exhibited the lowest albedo on Saturn in the CB2 channel. The region surrounding the dark region exhibited billowing cloud patterns that are similar to mid-latitudes of Jupiter. The storm also altered the zonal mean wind profile of the storm latitudes. Our cloud-tracking measurements reveal that the zonal mean cloud motions accelerated by 35~m$^{~}$s$^{-1}$ to the north of the storm around 38\degree N and decelerated by 30~m$^{~}$s$^{-1}$ to the south around 31\degree N. This change in the zonal wind speed is supported by the tropospheric warming detected by \cite{Achterberg_etal_2012DPS}. The warming is consistent with the latent heat released by the storm. Although the change in the cloud motion could be explained by changes in the cloud altitude and vertical shear, we believe that a real wind speed change was caused by the tropospheric warming. The westward jets at $\pm$35\degree~latitudes have often been the sites of storm activity. Saturn's 35\degree N region harbored several small cumulus storms during the Voyager flybys in 1980-81 \citep{Hunt_etal_1982, Sromovsky_etal_1983}. Earlier in the Cassini mission, similar behaviors, albeit at much smaller scales than the northern-hemisphere planet-encircling storms, were seen in the lightning storms observed in ``Storm Alley,'' at 35\degree S latitude. Like the new storm at 35\degree N, the cumulus events in Storm Alley drifted west and spawned anticyclonic ovals that slowly separated from the source region \citep{Porco_etal_2005, Dyudina_etal_2007}. Unlike in the new storm, the anticyclones drifted west relative to the source, and the source was intermittent. The area of the source region, intensity of the lightning activities, and the size of the anticyclonic vortices were an order of magnitude smaller in the southern storms than in the new northern storm. The five planet-encircling storms previously seen on Saturn alternated their location between equatorial and mid-latitudes \cite{Sanchez-Lavega_1994}. The last event of 1990 was equatorial \citep{Sanchez-Lavega_etal_1991, Sanchez-Lavega_1994, Beebe_etal_1992, Westphal_etal_1992}. The new storm of 2010-2011 followed the previous pattern of events by erupting in mid-latitude. Comparing the earlier storms observed from Earth with the unprecedented details of the present storm revealed by our present study is not straightforward. However, the large-scale structures seen by the ground-based observations of \cite{Sanchez-Lavega_etal_2012gws} are consistent with the results presented here, and give confidence in our ability to compare duration and the spatial structures of the present storm with the past ones. If we take December 5, 2010 as the beginning and June~20, 2011 as the end of the present storm, it lasted for 201~days, longer than any of the five previously recorded storms \citep{Sanchez-Lavega_1994}. Interestingly, the previous longest storm, which lasted for 150 days in 1903, erupted at 30$\pm$2\degree N, a latitude similar to the present storm. On Earth, one of the factors that contribute to the longevity of a thunderstorm is the lack of vertical shear \citep{Emanuel_1994}. The two long-lasting cumulus storms may indicate the lack of vertical shear at their latitudes. Episodic convective outbursts such as the Saturnian planet-circling storms suggest the presence of a mechanism that allows a large build-up of convective available potential energy (CAPE) between the large outbursts. At the depth of 10-bar at the predicted water condensation level, the static stability is believed to be enhanced by the latent heat release \citep{Weidenschilling_Lewis_1973, Sugiyama_etal_2006}. This layer of enhanced static stability could act as a lid for any convective activities that initiate underneath and store CAPE. An episodic cumulus storm can erupt when the internal heat flux deposits sufficient CAPE to break through the water condensation layer; this instability could be triggered spontaneously or modulated by seasonal effects. Perhaps the String of Pearls forms when Rossby waves are excited in the water condensation layer. \cite{Sayanagi_Showman_2007} demonstrated that Rossby waves excited at the 10-bar level can affect the cloud-top level. This possibility can be tested if simultaneous observations by VIMS and ISS reveal vertical offset in the SoPs phase; as those instruments sense different altitudes, comparing their images should reveal the vertical structure of the SoPs. If SoPs is indeed a Rossby wave, the ISS images should show SoPs phase shifted to the west compared to that in the VIMS images. This will be a topic for future investigation. Based on the records of past events, we expect the disturbance to have lasting effects on Saturn's northern hemisphere. As a point of comparison, the 1990 storm left the northern hemisphere of Saturn disturbed for the rest of the decade, and many activities were recorded until 1997 \citep{Sanchez-Lavega_etal_1993a, Sanchez-Lavega_etal_1996, Sanchez-Lavega_etal_1999}. The continuing disturbances of the 2010-2011 storm have been confirmed through ground-based telescopic observations and Cassini RPWS measurements. For example, in early April 2012, the RPWS detected electrostatic discharge signals emitted by a new storm. Cassini CIRS and ground-based infrared observations also continue to show lasting activities in the stratosphere \citep{Hesman_etal_2012DPS, Fletcher_etal_2012DPS, Hesman_etal_2012, Fletcher_etal_2012}, and show a large stratospheric hot spot that has been termed the~``beacon.'' Like the 1990 storm, the aftermath of the latest storm should have an effect that may last for up to a decade, and a continuing monitoring of Saturn from the orbiting vantage point of Cassini spacecraft should reveal further details of the dynamic event. \textbf{Acknowledgement:} Our work was supported by the Cassini-Huygens mission, a cooperative project of NASA, ESA, ASI, managed by JPL, a division of the California Institute of Technology, under a contract with NASA. The authors thank the two anonymous reviewers for their very constructive comments. \label{lastpage} \def\ref@jnl#1{{\jnl@style#1}}% \newcommand\aj{\ref@jnl{AJ}}% \newcommand\araa{\ref@jnl{ARA\&A}}% \newcommand\apj{\ref@jnl{ApJ}}% \newcommand\apjl{\ref@jnl{ApJ}}% \newcommand\apjs{\ref@jnl{ApJS}}% \newcommand\ao{\ref@jnl{Appl.~Opt.}}% \newcommand\apss{\ref@jnl{Ap\&SS}}% \newcommand\aap{\ref@jnl{A\&A}}% \newcommand\aapr{\ref@jnl{A\&A~Rev.}}% \newcommand\aaps{\ref@jnl{A\&AS}}% \newcommand\azh{\ref@jnl{AZh}}% \newcommand\baas{\ref@jnl{BAAS}}% \newcommand\jrasc{\ref@jnl{JRASC}}% \newcommand\memras{\ref@jnl{MmRAS}}% \newcommand\mnras{\ref@jnl{MNRAS}}% \newcommand\pra{\ref@jnl{Phys.~Rev.~A}}% \newcommand\prb{\ref@jnl{Phys.~Rev.~B}}% \newcommand\prc{\ref@jnl{Phys.~Rev.~C}}% \newcommand\prd{\ref@jnl{Phys.~Rev.~D}}% \newcommand\pre{\ref@jnl{Phys.~Rev.~E}}% \newcommand\prl{\ref@jnl{Phys.~Rev.~Lett.}}% \newcommand\pasp{\ref@jnl{PASP}}% \newcommand\pasj{\ref@jnl{PASJ}}% \newcommand\qjras{\ref@jnl{QJRAS}}% \newcommand\skytel{\ref@jnl{S\&T}}% \newcommand\solphys{\ref@jnl{Sol.~Phys.}}% \newcommand\sovast{\ref@jnl{Soviet~Ast.}}% \newcommand\ssr{\ref@jnl{Space~Sci.~Rev.}}% \newcommand\zap{\ref@jnl{ZAp}}% \newcommand\nat{\ref@jnl{Nature}}% \newcommand\iaucirc{\ref@jnl{IAU~Circ.}}% \newcommand\aplett{\ref@jnl{Astrophys.~Lett.}}% \newcommand\apspr{\ref@jnl{Astrophys.~Space~Phys.~Res.}}% \newcommand\bain{\ref@jnl{Bull.~Astron.~Inst.~Netherlands}}% \newcommand\fcp{\ref@jnl{Fund.~Cosmic~Phys.}}% \newcommand\gca{\ref@jnl{Geochim.~Cosmochim.~Acta}}% \newcommand\grl{\ref@jnl{Geophys.~Res.~Lett.}}% \newcommand\jcp{\ref@jnl{J.~Chem.~Phys.}}% \newcommand\jgr{\ref@jnl{J.~Geophys.~Res.}}% \newcommand\jqsrt{\ref@jnl{J.~Quant.~Spec.~Radiat.~Transf.}}% \newcommand\memsai{\ref@jnl{Mem.~Soc.~Astron.~Italiana}}% \newcommand\nphysa{\ref@jnl{Nucl.~Phys.~A}}% \newcommand\physrep{\ref@jnl{Phys.~Rep.}}% \newcommand\physscr{\ref@jnl{Phys.~Scr}}% \newcommand\planss{\ref@jnl{Planet.~Space~Sci.}}% \newcommand\procspie{\ref@jnl{Proc.~SPIE}}% \let\astap=\aap \let\apjlett=\apjl \let\apjsupp=\apjs \let\applopt=\ao | 16 | 7 | 1607.07246 | Saturn’s quasi-periodic planet-encircling storms are the largest convecting cumulus outbursts in the Solar System. The last eruption was in 1990 (Sánchez-Lavega, A. [1994]. Chaos 4, 341-353). A new eruption started in December 2010 and presented the first-ever opportunity to observe such episodic storms from a spacecraft in orbit around Saturn (Fischer, G. et al. [2011]. Nature 475, 75-77; Sánchez-Lavega, A. et al. [2011]. Nature 475, 71-74; Fletcher, L.N. et al. [2011]. Science 332, 1413). Here, we analyze images acquired with the Cassini Imaging Science Subsystem (ISS), which captured the storm’s birth, evolution, and demise. In studying the end of the convective activity, we also analyze the Saturn Electrostatic Discharge (SED) signals detected by the Radio and Plasma Wave Science (RPWS) instrument. The storm’s initial position coincided with that of a previously known feature called the String of Pearls (SoPs) at 33°N planetocentric latitude. Intense cumulus convection at the westernmost point of the storm formed a particularly bright “head” that drifted at -26.9 ± 0.8 m s<SUP>-1</SUP> (negative denotes westward motion). On January 11, 2011, the size of the head was 9200 km and up to 34,000 km in the north-south and east-west dimensions, respectively. RPWS measurements show that the longitudinal extent of the lightning source expanded with the storm’s growth. The storm spawned the largest tropospheric vortex ever seen on Saturn. On January 11, 2011, the anticyclone was sized 11,000 km by 12,000 km in the north-south and east-west directions, respectively. Between January and September 2011, the vortex drifted at an average speed of -8.4 m s<SUP>-1</SUP>. We detect anticyclonic circulation in the new vortex. The vortex’s size gradually decreased after its formation, and its central latitude shifted to the north. The storm’s head moved westward and encountered the new anticyclone from the east in June 2011. After the head-vortex collision, the RPWS instrument detected that the SED activities became intermittent and declined over ∼40 days until the signals became undetectable in early August. In late August, the SED radio signals resurged for 9 days. The storm left a vast dark area between 32°N and 38°N latitudes, surrounded by a highly disturbed region that resembles the mid-latitudes of Jupiter. Using ISS images, we also made cloud-tracking wind measurements that reveal differences in the cloud-level zonal wind profiles before and after the storm. | false | [
"such episodic storms",
"early August",
"G. et al",
"∼40 days",
"L.N. et al",
"A. et al",
"late August",
"Plasma Wave Science",
"SED",
"Jupiter",
"Saturn",
"the SED radio signals",
"motion",
"demise",
"latitudes",
"Saturn’s quasi-periodic planet-encircling storms",
"RPWS",
"-",
"m",
"Intense cumulus convection"
] | 8.50624 | 15.511942 | 96 |
12408831 | [
"Leggett, S. K.",
"Cushing, Michael C.",
"Hardegree-Ullman, Kevin K.",
"Trucks, Jesica L.",
"Marley, M. S.",
"Morley, Caroline V.",
"Saumon, D.",
"Carey, S. J.",
"Fortney, J. J.",
"Gelino, C. R.",
"Gizis, J. E.",
"Kirkpatrick, J. D.",
"Mace, G. N."
] | 2016ApJ...830..141L | [
"Observed Variability at 1 and 4 μm in the Y0 Brown Dwarf WISEP J173835.52+273258.9"
] | 31 | [
"Gemini Observatory, Northern Operations Center, 670 N. A'ohoku Place, Hilo, HI 96720, USA",
"The University of Toledo, 2801 West Bancroft Street, Mailstop 111, Toledo, OH 43606, USA",
"The University of Toledo, 2801 West Bancroft Street, Mailstop 111, Toledo, OH 43606, USA",
"The University of Toledo, 2801 West Bancroft Street, Mailstop 111, Toledo, OH 43606, USA",
"NASA Ames Research Center, Mail Stop 245-3, Moffett Field, CA 94035, USA",
"Department of Astronomy and Astrophysics, University of California, Santa Cruz, CA 95064, USA",
"Los Alamos National Laboratory, P.O. Box 1663, MS F663, Los Alamos, NM 87545, USA",
"Spitzer Science Center, CalTech, Pasadena, CA 91125, USA",
"Department of Astronomy and Astrophysics, University of California, Santa Cruz, CA 95064, USA",
"IPAC, CalTech, Pasadena, CA 91125, USA ; NASA Exoplanet Science Institute, CalTech, Pasadena, CA 91125, USA",
"University of Delaware, Newark, DE 19716, USA",
"IPAC, CalTech, Pasadena, CA 91125, USA",
"University of Texas, Austin, TX 78712, USA"
] | [
"2016ApJ...832...58E",
"2017ApJ...842...78V",
"2017ApJ...842..118L",
"2017MNRAS.468.3764S",
"2017arXiv170802821A",
"2018AJ....155..238S",
"2018ApJ...867...96L",
"2018ApJ...867..109M",
"2018haex.bookE..94A",
"2019A&A...629A.145E",
"2019ApJ...874..111T",
"2019ApJ...875L..15M",
"2019ApJ...877...24Z",
"2019ApJ...882..117L",
"2019ApJ...883....4S",
"2019MNRAS.487.1994K",
"2020AJ....160...38V",
"2020NatAs...4..453D",
"2021AJ....161..224T",
"2021ApJ...918...11L",
"2021ApJ...918L..25L",
"2021JGRE..12606655G",
"2021MNRAS.502..678T",
"2021MNRAS.502.2198T",
"2021arXiv210700696L",
"2022ApJ...924...68V",
"2022ApJ...928..166L",
"2023MNRAS.520.4235S",
"2024A&A...686A..73M",
"2024MNRAS.527.6624L",
"2024MNRAS.531.2168S"
] | [
"astronomy"
] | 10 | [
"brown dwarfs",
"stars: atmospheres",
"stars: individual: WISEP J173835.52+273258.9",
"Astrophysics - Solar and Stellar Astrophysics"
] | [
"1989AJ.....98.2268H",
"1997ApJ...491..856B",
"2000ASPC..212.....G",
"2003AJ....126..348T",
"2003AJ....126.1006E",
"2003ApJ...596..587B",
"2003PASP..115.1388H",
"2004ApJS..154...10F",
"2008A&A...487..277G",
"2008ApJ...689.1327S",
"2009ApJ...701.1534A",
"2010ApJ...710.1142B",
"2010arXiv1008.4686H",
"2011ApJ...730L...9L",
"2011ApJ...743...50C",
"2011MNRAS.417.2874M",
"2012ApJ...750..105R",
"2012ApJ...753..156K",
"2012ApJ...756..172M",
"2012ApJ...758...57L",
"2012ApJ...759...60T",
"2012Icar..220....6S",
"2013ApJ...763..130L",
"2013ApJ...776...85S",
"2014A&A...566A.111W",
"2014A&A...566A.130C",
"2014AJ....147..113C",
"2014ApJ...783...68B",
"2014ApJ...785..158R",
"2014ApJ...786L..18L",
"2014ApJ...787...78M",
"2014ApJ...793...75R",
"2014ApJ...797..120R",
"2014MNRAS.444.1931P",
"2015ApJ...799...37L",
"2015ApJ...799..154M",
"2015ApJ...801..104H",
"2015ApJ...804...92S",
"2015ApJ...804L..17T",
"2015MNRAS.448.3775R",
"2016ApJ...817..162S",
"2016ApJ...818..176Z",
"2016ApJ...823..152C",
"2016ApJ...824....2L",
"2016ApJ...826....8Y"
] | [
"10.3847/0004-637X/830/2/141",
"10.48550/arXiv.1607.07888"
] | 1607 | 1607.07888_arXiv.txt | There are now more than twenty brown dwarfs known in the solar neighborhood that have effective temperatures ($T_{\rm eff}$) lower than 500~K (Cushing et al. 2011, 2014; Kirkpatrick et al. 2012; Liu et al. 2012; Luhman 2014; Luhman, Burgasser \& Bochanski 2011, Pinfield et al. 2014; Schneider et al. 2015; Tinney et al. 2012). These have been classified as Y dwarfs (Cushing et al. 2011, Kirkpatrick et al. 2012). Evolutionary models show that for $300 \leq T_{\rm eff}$~K $\leq 500$ and 0.2 $\leq$ age~Gyr $\leq$ 10 (as appropriate for the solar neighborhood) the mass range is 2 -- 30 Jupiter masses (Saumon \& Marley 2008). Hence this population of isolated brown dwarfs has a mass that is very planet-like. Our group has an ongoing program measuring the photometric variability of Y dwarfs. For warmer brown dwarfs variability is usually associated with inhomogeneous or variable cloud structure in the atmosphere (e.g. Radigan et al. 2012). For Y0 and Y1 dwarfs with $T_{\rm eff} \approx 400$~K the atmospheres are generally cloud-free, because most of the atmosphere is too cold for chloride or sulphide clouds, and too warm for water or ammonia clouds (Burrows et al. 2003; Morley et al. 2012, 2014); in fact cloud-free models can reproduce Y dwarf observations (Leggett et al. 2015, 2016). Nevertheless variability may be seen at wavelengths where flux is emitted from very high and cold or low and warm layers where condensates can be present (Morley et al. 2012), or variability may be seen due to temperature variations across the brown dwarf surface (Showman \& Kaspi 2013). In our first paper (Cushing et al. 2016, hereafter Cu16) we show that the Y0.5(pec) brown dwarf WISEPC J140518.40$+$553421.5 (W1405, Cushing et al. 2011) is variable at mid-infrared wavelengths. W1405 was observed with {\em Spitzer} using the IRAC camera (Fazio et al. 2004) in the [3.6] and [4.5] filters. Variability was evident at [4.5] in the first epoch and at both [3.6] and [4.5] in the second epoch. The second-epoch light curves have a period of about 8.5 hr, and semi-amplitudes of 3.5\%. In the current paper we present the detection of variability at mid-infrared wavelengths in another Y0, WISEP J173835.52$+$273258.9 (W1738, Cushing et al. 2011). We also present the tentative detection of variability at near-infrared wavelengths, at the $\lesssim 2 \sigma$ confidence level. We extend to lower limits the work of Rajan et al. (2015) who excluded any $J$-band variability larger than 20\% for this brown dwarf. Leggett et al. (2016) compares near-infrared spectra and photometry for W1738 to recent models which include chemical disequilibrium driven by vertical transport (Tremblin et al. 2015). It was necessary to include mixing in order to reproduce the observations, and a cloud-free solar metallicity model with $T_{\rm eff} = 425 \pm 25$K and log $g = 4.0 \pm 0.25$ fit the data best. This temperature and relatively low gravity imply that W1738 is a 3 -- 9 Jupiter mass object with an age of 0.15 -- 1 Gyr. Table 1 lists properties of W1738. In \S 2 we present new observations of W1738 obtained with {\em Spitzer} and IRAC, and Gemini Observatory and its near-infrared imager NIRI (Hodapp et al. 2003). We obtained two epochs of [3.6] and [4.5] data, separated by four months, as well as two epochs of near-infrared $Y$ and $J$ data, obtained between the {\em Spitzer} observations and separated by one month. \S 3 presents our analysis of the data, which we discuss in \S 4. Our conclusions are given in \S 5. | We obtained 12 hours of continuous {\em Spitzer} data on the Y0 brown dwarf W1738 at [3.6], followed by another 12 hours at [4.5]. Two sets of data were obtained four months apart, on June 30 and October 30 2013. We also obtained interspersed Gemini $Y$ and $J$ data on W1738, with about 1.4 hours on-source at $Y$ and 2.3 hours on-source at $J$, on two occasions separated by about one month, July 17 and August 23 2013. Fourier and Lomb-Scargle analyses of the mid-infrared [4.5] data suggested the presence of three and six hour periods in the data. We use a probabilistic method to fit double sinusoids to the [4.5] data (the near-infrared data covers a short time span and the [3.6] data are too noisy). We constrain the second sinusoid to have half the period of the first, but allow amplitude and phase to vary. We find sinusoids with periods of 5.8 $\pm$ 0.1 hours and 6.13 $\pm$ 0.08 hours, and half those values, reproduce the observed [4.5] light curves on the two epochs well. The amplitudes range from 0.3\% to 1.1\%, leading to peak-to-peak variability of 3\%. The shorter time-span near-infrared data was inspected visually only. The data suggest that W1738 is also variable in the near-infrared, although only at the $\lesssim 2 \sigma$ confidence level. If real, the implication is that this Y0 dwarf varied by 10 -- 30\% in $Y$ and 5 -- 15 \% in $J$, peak-to-peak, with a period of about three hours, at two epochs during 2013. The observations are consistent with W1738, a 5 Jupiter-mass $T_{\rm eff} \approx 400$ K Y-type brown dwarf, being seen nearly-equator on, having a rotation period of $6.0 \pm 0.1$ hours, and having one or more large surface features which give rise to the variability. The features evolve over timescales of months. The observed variability at $\lambda \sim 4\,\mu$m is likely due to thermal variations caused by atmospheric circulation, while the larger variations at $\lambda \sim 1\,\mu$m, if real, may be due to the presence of patchy clouds of KCl and Na$_2$S in the lower regions of the atmosphere. | 16 | 7 | 1607.07888 | We have monitored photometrically the Y0 brown dwarf WISEP J173835.52+273258.9 (W1738) at both near- and mid-infrared wavelengths. This ≲1 Gyr old 400 K dwarf is at a distance of 8 pc and has a mass around 5 M <SUB>Jupiter</SUB>. We observed W1738 using two near-infrared filters at λ ≈ 1 μm, Y and J, on Gemini Observatory and two mid-infrared filters at λ ≈ 4 μm, [3.6] and [4.5], on the Spitzer observatory. Twenty-four hours were spent on the source by Spitzer on each of 2013 June 30 and October 30 UT. Between these observations, around 5 hr were spent on the source by Gemini on each of 2013 July 17 and August 23 UT. The mid-infrared light curves show significant evolution between the two observations separated by 4 months. We find that a double sinusoid can be fit to the [4.5] data, where one sinusoid has a period of 6.0 ± 0.1 hr and the other a period of 3.0 ± 0.1 hr. The near-infrared observations suggest variability with a ∼3.0 hr period, although only at a ≲2σ confidence level. We interpret our results as showing that the Y dwarf has a 6.0 ± 0.1 hr rotation period, with one or more large-scale surface features being the source of variability. The peak-to-peak amplitude of the light curve at [4.5] is 3%. The amplitude of the near-infrared variability, if real, may be as high as 5%-30%. Intriguingly, this size of variability and the wavelength dependence can be reproduced by atmospheric models that include patchy KCl and Na<SUB>2</SUB>S clouds and associated small changes in surface temperature. The small number of large features, as well as the timescale for evolution of the features, is very similar to what is seen in the atmospheres of the solar system gas giants. | false | [
"large features",
"associated small changes",
"surface temperature",
"-infrared wavelengths",
"significant evolution",
"patchy KCl",
"atmospheric models",
"Jupiter</SUB",
"variability",
"evolution",
"The mid-infrared light curves",
"the solar system gas giants",
"a ∼3.0 hr period",
"Gemini Observatory",
"two mid-infrared filters",
"WISEP J173835.52",
"Gemini",
"October",
"a ≲2σ confidence level",
"Na<SUB>2</SUB>S clouds"
] | 7.209815 | 12.589618 | 102 |
12518466 | [
"Bentum, Mark J.",
"Bonetti, Luca",
"Spallicci, Alessandro D. A. M."
] | 2017AdSpR..59..736B | [
"Dispersion by pulsars, magnetars, fast radio bursts and massive electromagnetism at very low radio frequencies"
] | 28 | [
"Universiteit van Twente, Faculteit van Elektrotechniek, Wiskunde en Informatica, Telecommunication Engineering Postbus 217, 7500 AE Enschede, The Netherlands; ASTRON, Postbus 2, 7900 AA Dwingeloo, The Netherlands",
"Université d'Orléans, Observatoire des Sciences de l'Univers en région Centre, 1A rue de la Férollerie, 45071 Orléans, France; Université d'Orléans, Collegium Sciences et Techniques, Pôle de Physique, Rue de Chartres, 45100 Orléans, France",
"Université d'Orléans, Observatoire des Sciences de l'Univers en région Centre, 1A rue de la Férollerie, 45071 Orléans, France; Université d'Orléans, Collegium Sciences et Techniques, Pôle de Physique, Rue de Chartres, 45100 Orléans, France"
] | [
"2017ApJ...842...23Y",
"2017CQGra..34u5006S",
"2017FoPh...47..769A",
"2017PhLB..764..203B",
"2017PhLB..768..326B",
"2017RAA....17...13W",
"2018EPJC...78..811B",
"2018JCAP...07..045W",
"2018PhRvD..97l4016C",
"2019EPJC...79..590H",
"2019PhRvD..99l4001C",
"2019arXiv191206288S",
"2020RAA....20..206W",
"2021EPJC...81....4S",
"2021FrPhy..1644300W",
"2021PhRvD.103d4008M",
"2021PhRvD.104j3516B",
"2022EPJP..137..253S",
"2022EPJP..137.1386S",
"2022FoPh...52...23S",
"2022IJMPA..3750132K",
"2022PhRvD.106k6014M",
"2022arXiv220502487S",
"2023JCAP...09..025W",
"2023MNRAS.520.1324L",
"2023PhRvD.107k5018M",
"2023arXiv231101899N",
"2024ChPhL..41e9501R"
] | [
"astronomy",
"physics"
] | 6 | [
"Photons",
"Radio astronomy",
"Satellites",
"Pulsars",
"Magnetars",
"Fast radio bursts",
"Astrophysics - Instrumentation and Methods for Astrophysics",
"Astrophysics - High Energy Astrophysical Phenomena",
"General Relativity and Quantum Cosmology",
"High Energy Physics - Phenomenology"
] | [
"1933PhRv...44..855H",
"1934PhRv...46.1087B",
"1934RSPSA.144..425B",
"1936AnP...418..398E",
"1936ZPhy...98..714H",
"1940AnP...430..345B",
"1941PhRv...60..121L",
"1941PhRv...60..514L",
"1942PhRv...62...68P",
"1944PhRv...65..175L",
"1944PhRv...65..228P",
"1948RvMP...20...40P",
"1950PhRv...80..380K",
"1951PhRv...83..776K",
"1959PThPS..11....1Y",
"1967NCimB..50..371S",
"1969Sci...166..879F",
"1971PhRvL..26..721W",
"1971RvMP...43..277G",
"1972PhRvD...5..796B",
"1975PhRvL..35.1117B",
"1976SvPhU..19..624C",
"1980panp.book.....M",
"1985SvAL...11...39S",
"1986SvAL...12..325K",
"1988SvAL...14..181S",
"1989PhRvA..40..579W",
"1989PhRvA..40..588F",
"1991ApJ...373L..17H",
"1992ApJ...385..273P",
"1997CQGra..14.2971S",
"1997PPCF...39...73R",
"1997PhRvL..79.1626B",
"1999CQGra..16.1313B",
"1999PhRvL..82.4964S",
"2002IJMPD..11...35A",
"2002PhLB..539..126K",
"2003AdSpR..31.2461D",
"2003PhRvD..67b4015C",
"2003PhRvD..67d4017M",
"2005CQGra..22S.461S",
"2005ChPhL..22.3057T",
"2005MNRAS.357.1013A",
"2005RPPh...68...77T",
"2006Sci...314...97K",
"2007ApJ...668L.143D",
"2007MNRAS.377..677A",
"2007PPCF...49..429R",
"2007PhRvL..98a0402A",
"2008ApJ...684..870K",
"2009Natur.462..291A",
"2009Natur.462..331A",
"2010IJMPA..25.5409M",
"2010MNRAS.406..863L",
"2010PhRvD..82f5026A",
"2010RvMP...82..939G",
"2011AcAau..68.1392V",
"2011AdSpR..47..380B",
"2011AdSpR..47..645T",
"2011EPJD...61..531S",
"2012A&A...543A..66H",
"2013A&A...556A...2V",
"2013APh....43...50E",
"2013ApJ...764..187S",
"2013ApJ...776L..16T",
"2013CQGra..30v4006S",
"2013CQGra..30v4011L",
"2013ITAP...61.2540E",
"2013PASA...30...31B",
"2014AcAau.102..321D",
"2014ApJ...786...84K",
"2014ChPhC..38i0001O",
"2014LRR....17....4W",
"2014MNRAS.441L..26T",
"2014MNRAS.443L..11D",
"2014PhRvD..90d4048N",
"2015A&A...579A..69O",
"2015AJ....150..199T",
"2015AcAau.107...14B",
"2015AdSpR..55..501A",
"2015ApJ...801..130L",
"2015ApJ...808...81P",
"2015ApJ...815...89P",
"2015GReGr..47....5K",
"2015JPhA...48Q5401G",
"2015MNRAS.449.2685C",
"2015MNRAS.451L..50T",
"2015MNRAS.452.1254K",
"2015PhRvD..92b5039S",
"2016A&A...586A..92P",
"2016APh....82...49R",
"2016ApJ...817...16C",
"2016ApJ...822L..15W",
"2016ExA....41..271R",
"2016MNRAS.458.3506R",
"2016MPLA...3130013K",
"2016Natur.530..453K",
"2016PASA...33...45P",
"2016PhLB..757..548B",
"2016PhRvD..93f3521S",
"2016PhRvD..94b4061W",
"2016PhRvL.116f1102A",
"2017PhLB..764..203B"
] | [
"10.1016/j.asr.2016.10.018",
"10.48550/arXiv.1607.08820"
] | 1607 | 1607.08820_arXiv.txt | For many years, many of us have worked, see {\it e.g.}, \cite{spalliccietal2005}, for the opening of one of the gravitational wave windows on the universe, that has finally occurred \cite{abbottetal2016}, while for other windows we still have to wait \cite{spallicci2013}. Nevertheless, we can genuinely state that even when gravitational wave information will be exploited by ground or space laser interferometers, or by the pulsar timing array, our understanding of the large scale universe will be largely (exception made for neutrinos and cosmic rays) based on electromagnetic observations of the four interactions that rule the physical world. As photons are the messengers, fundamental physics has the concern of testing the foundations of electromagnetism, while astrophysics the task of interpreting the universe accordingly. { Furthermore, while alternative theories to general relativity, including those based on massive gravitons, are also conceived for solving the questions raised by the dark universe or to couple gravity with the other interactions, less effort is deployed for studying alternative electromagnetism. But electromagnetism at large scales may differ from the Maxwellian conception of the nineteenth century and thereby contribute to solve some of the riddles in contemporary physics and cosmology}. Since neutrinos have been declared massive, while waiting for the elusive graviton, and leaving aside gluons that are not observed as free particles, the photon is the sole particle of the standard model to be massless. Finally, massive photons would manifest themselves through delays of low frequency electromagnetic signals, being the sub-MHz part of the spectrum yet unexplored \cite{lacki2010}. Thus, this work addresses the potential of the low frequency region to test the foundations of physics. We shall use SI units throughout the paper. | We have focused on the interest raised by the newly operating and future ground and space detectors at very low radio frequencies. The foundations of electromagnetism could be tested, confirming our beliefs or else contributing to establish new physics. Studies on dispersion appear crucial to unveil the causes of the excess of DM, and disentangle the measure of DM from distance. Low frequency observatories placed faraway from the Earth will be an essential aid to such studies, and will possibly set competitive limits to the photon mass. Meanwhile, theoretical investigations on the plausibility and implications of massive photons is to be pursued. In the context of Standard Model Extensions (SMEs), four general classes of Super Symmetry (SuSy) and Lorentz Symmetry (LoSy) breaking were analysed, leading to observable imprints at our energy scales \cite{bodshnsp2016a}. The photon dispersion relations show a non-Maxwellian behaviour for the CPT (Charge-Parity-Time reversal symmetry) odd and even sectors. The group velocities exhibit also a directional dependence with respect to the breaking background vector (odd CPT) or tensor (even CPT). In the former sector, the group velocity may decay following an inverse squared frequency behaviour. Thus, a massive and gauge invariant Carroll-Field-Jackiw photon term in the Lagrangian has been extracted and the induced mass shown to be proportional to the breaking vector. The latter is estimated by ground measurements and leads to a photon mass upper limit of $10^{-19}$ eV or $2 \times 10^{-55}$ kg. Implications for cosmology by non-linear and massive photon theories, and generally non-Maxwellian behaviour have not yet been evaluated adequately. Finally, laboratory experiments should be pursued, investigating in all directions, including the search of frequency shifts, incidentally using the same equipment to set upper limit to the Hubble parameter at small scale \cite{shamirfox1967,bonnor1999,dumin2012,priceromano2012,kopeikin2015}. | 16 | 7 | 1607.08820 | Our understanding of the universe relies mostly on electromagnetism. As photons are the messengers, fundamental physics is concerned in testing their properties. Photon mass upper limits have been earlier set through pulsar observations, but new investigations are offered by the excess of dispersion measure (DM), sometimes observed with pulsar and magnetar data at low frequencies, or with the fast radio bursts (FRBs), of yet unknown origin. Arguments for the excess of DM do not reach a consensus, but are not mutually exclusive. Thus, we remind that for massive electromagnetism, dispersion goes as the inverse of the frequency squared. Thereby, new avenues are offered also by the recently operating ground observatories in 10-80 MHz domain and by the proposed Orbiting Low Frequency Antennas for Radio astronomy (OLFAR). The latter acts as a large aperture dish by employing a swarm of nano-satellites observing the sky for the first time in the 0.1-15 MHz spectrum. The swarm must be deployed sufficiently away from the ionosphere to avoid distorsions from terrestrial interference, especially during solar maxima, and offer stable conditions for calibration during observations. | false | [
"unknown origin",
"pulsar observations",
"Radio astronomy",
"OLFAR",
"observations",
"low frequencies",
"new investigations",
"dispersion measure",
"stable conditions",
"the proposed Orbiting Low Frequency Antennas",
"solar maxima",
"new avenues",
"dispersion",
"terrestrial interference",
"FRBs",
"calibration",
"massive electromagnetism",
"DM",
"electromagnetism",
"Photon mass upper limits"
] | 6.506097 | 3.84499 | -1 |
12406228 | [
"Eistrup, Christian",
"Walsh, Catherine",
"van Dishoeck, Ewine F."
] | 2016A&A...595A..83E | [
"Setting the volatile composition of (exo)planet-building material. Does chemical evolution in disk midplanes matter?"
] | 146 | [
"Leiden Observatory, Leiden University, PO Box 9513, 2300 RA, Leiden, The Netherlands",
"Leiden Observatory, Leiden University, PO Box 9513, 2300 RA, Leiden, The Netherlands ; School of Physics and Astronomy, University of Leeds, Leeds, LS2 9JT, UK",
"Leiden Observatory, Leiden University, PO Box 9513, 2300 RA, Leiden, The Netherlands ; Max-Planck-Institut für Extraterrestrische Physik, Giessenbachstrasse 1, 85748, Garching, Germany"
] | [
"2017A&A...599A.101V",
"2017A&A...599A.113M",
"2017A&A...601A..36B",
"2017A&A...603A.123H",
"2017A&A...605A..69T",
"2017A&A...607A.114W",
"2017AJ....153..240A",
"2017ApJ...836..118N",
"2017MNRAS.469.3910C",
"2017MNRAS.469.3994B",
"2017MNRAS.472..189I",
"2017MNRAS.472..447M",
"2018A&A...611A..80B",
"2018A&A...612A..88L",
"2018A&A...613A..14E",
"2018A&A...613A..29V",
"2018A&A...615A..83V",
"2018A&A...618A.182B",
"2018ApJ...852...18T",
"2018ApJ...852..122H",
"2018ApJ...855...62N",
"2018ApJ...856...85S",
"2018ApJ...859..131L",
"2018ApJ...865..155C",
"2018ApJ...866...46M",
"2018ApJ...868L..37D",
"2018IAUS..332...57C",
"2018IAUS..332...69E",
"2018IAUS..332...88V",
"2018IAUS..332..113N",
"2018IAUS..332..124M",
"2018MNRAS.473..757B",
"2018cwla.conf...89K",
"2018exha.book.....P",
"2019A&A...621A..75E",
"2019A&A...627A.127C",
"2019A&A...629A..64B",
"2019A&A...629A..84E",
"2019A&A...631A..69M",
"2019A&A...632A..63C",
"2019A&A...632L..10B",
"2019ARA&A..57..113A",
"2019ARA&A..57..617M",
"2019AREPS..47..583H",
"2019ApJ...870..129W",
"2019ApJ...874...92P",
"2019ApJ...876...25B",
"2019ApJ...876L..13S",
"2019ApJ...877...24Z",
"2019ApJ...877..131S",
"2019ApJ...883...98Z",
"2019ApJ...885..146R",
"2019MNRAS.484..345C",
"2019MNRAS.486.4638U",
"2019MNRAS.487.3998B",
"2020A&A...633A..10B",
"2020A&A...633A.110G",
"2020A&A...635A..68C",
"2020A&A...640A.131M",
"2020A&A...642A.229C",
"2020AJ....160..248A",
"2020ApJ...890..154P",
"2020ApJ...891L..16Z",
"2020ApJ...891L..17Z",
"2020ApJ...901..166V",
"2020ApJ...904..129P",
"2020ApJ...905...68A",
"2020IAUS..345..115K",
"2020IAUS..350...91P",
"2020IAUS..350..207K",
"2020M&PS...55..575V",
"2020MNRAS.493..661D",
"2020MNRAS.499.2229N",
"2020SPIE11446E..0OL",
"2020arXiv200808106V",
"2021A&A...646A...3L",
"2021A&A...648A..73B",
"2021A&A...650A.168P",
"2021A&A...650A.180N",
"2021A&A...651A..48M",
"2021A&A...654A..71S",
"2021A&A...656A..90K",
"2021AJ....162...73P",
"2021AJ....162...74L",
"2021AJ....162...99F",
"2021ApJ...909...40T",
"2021ApJ...909...55A",
"2021ApJ...914...12L",
"2021ApJS..257....5Z",
"2021ApJS..257....7B",
"2021MNRAS.500.4658M",
"2021PASA...38...55K",
"2021arXiv210404824T",
"2021arXiv211008286V",
"2022A&A...658A..72P",
"2022A&A...665A..68M",
"2022A&A...665A.138B",
"2022A&A...666A..35T",
"2022A&A...666L...8S",
"2022A&A...667A.121E",
"2022A&A...667A.160E",
"2022ApJ...924....5V",
"2022ApJ...925...49R",
"2022ApJ...932....6V",
"2022ApJ...932...20D",
"2022ApJ...933L..40B",
"2022ApJ...934...74M",
"2022ApJ...936...44Z",
"2022ApJ...936..188N",
"2022ApJ...937...36P",
"2022ApJ...937L..37F",
"2022ApJ...938...29F",
"2022ExA....53..225T",
"2022ExA....53..323H",
"2022MNRAS.510.1148S",
"2022MNRAS.517.2285C",
"2022NatAs...6..635B",
"2022arXiv220408622V",
"2022arXiv220504100G",
"2023A&A...670A..12S",
"2023A&A...674A.211C",
"2023A&A...678A..33J",
"2023A&A...679A..55T",
"2023AJ....166..192A",
"2023ARA&A..61..287O",
"2023ASPC..534..947G",
"2023ApJ...943..112C",
"2023ApJ...946...18O",
"2023ApJ...947....7B",
"2023ApJ...953..183P",
"2023ApJ...956..125O",
"2023ESC.....7..260E",
"2023MNRAS.520.4683F",
"2023MNRAS.523.4365W",
"2023Natur.614..670F",
"2023arXiv230208317T",
"2023arXiv230408104G",
"2024A&A...686A.127B",
"2024AJ....167...66Y",
"2024ApJ...962...90D",
"2024NatAs...8..587F",
"2024arXiv240415555M",
"2024arXiv240604457R",
"2024arXiv240611470R",
"2024arXiv240612393D",
"2024arXiv240616498F"
] | [
"astronomy"
] | 12 | [
"astrochemistry",
"planets and satellites: formation",
"protoplanetary disks",
"planets and satellites: atmospheres",
"molecular processes",
"Astrophysics - Earth and Planetary Astrophysics"
] | [
"1972GeCoA..36..597G",
"1977Ap&SS..51..153W",
"1982A&A...114..245T",
"1983ApJ...267..603P",
"1993MNRAS.261...83H",
"1998A&A...338..995W",
"1999A&A...351..233A",
"1999ApJ...519..705A",
"2004A&A...417...93S",
"2004A&A...421.1075D",
"2004ApJ...604..388I",
"2004ApJS..151...35G",
"2005A&A...434..971D",
"2005A&A...438..923N",
"2007ARA&A..45..397U",
"2007ApJ...660..441W",
"2007prpl.conf..555D",
"2007prpl.conf..751B",
"2008ApJ...672..629V",
"2008ApJ...673..487I",
"2008ApJ...682..283G",
"2009A&A...495..881V",
"2009A&A...501..383W",
"2009ApJ...690...69U",
"2009ApJ...690.1497H",
"2010ARA&A..48...47A",
"2010ARA&A..48..631S",
"2010ApJ...722.1607W",
"2010ApJ...723.1241A",
"2010FaDi..147..509M",
"2010Natur.465.1049S",
"2011ARA&A..49...67W",
"2011ARA&A..49..195A",
"2011ARA&A..49..471M",
"2011ApJ...732..106F",
"2011ApJ...736...19B",
"2011ApJ...740..109O",
"2011ApJ...743L..16O",
"2012ApJ...747..114W",
"2012ApJ...757..192J",
"2013A&A...550A..36M",
"2013A&A...558A.109A",
"2013A&A...559A..24K",
"2013A&A...559A..46B",
"2013ApJ...763...25M",
"2013ApJ...772....5C",
"2013ApJ...777...28C",
"2013ApJS..204...24B",
"2013MNRAS.428.3262N",
"2013MNRAS.436L..35B",
"2014A&A...562A..26B",
"2014A&A...570A..35M",
"2014ApJ...790...97F",
"2014ApJ...794..123C",
"2014ApJ...797..113S",
"2014Life....4..142H",
"2014Natur.513..526F",
"2014Sci...345.1590C",
"2014prpl.conf..363P",
"2014prpl.conf..547J",
"2014prpl.conf..691B",
"2014prpl.conf..715F",
"2014prpl.conf..859C",
"2015A&A...574A.138T",
"2015A&A...577A.102V",
"2015A&A...579A..82R",
"2015A&A...582A..88W",
"2015ARA&A..53..541B",
"2015ApJ...806L...7Z",
"2015ApJ...807L..32D",
"2015ApJ...808L...3A",
"2015ApJ...815..109P",
"2015ApJ...815L..11R",
"2015Natur.526..678B",
"2015PASP..127..941C",
"2016A&A...588A..72W",
"2016A&A...588A.108K",
"2016A&A...592A..83K",
"2016ApJ...818...16M",
"2016ApJ...819L...7N",
"2016ApJ...823...91S",
"2016Natur.529...59S"
] | [
"10.1051/0004-6361/201628509",
"10.48550/arXiv.1607.06710"
] | 1607 | 1607.06710_arXiv.txt | \label{intr} The discovery of more than 2000 extrasolar planets by the radial velocity and transiting techniques \citep[e.g.,][]{udry2007,borucki2011,batalha2013,fischer2014} has signaled the next phase in exoplanet research: the characterization of their atmospheres. Simple molecules such as CO, \ce{H2O} and perhaps \ce{CO2} and \ce{CH4} are being detected in a growing number of exoplanet atmospheres \citep[e.g.,][]{seager2010,snellen2010,birkby2013,fraine2014,crossfield2015,sing2016}. These atmospheres are thought to be built up largely by the accretion of pebbles and planetesimals in the natal protoplanetary disk (see \citealt{johansen2014} and \citealt{benz2014} for reviews), hence the atmospheres should reflect the chemical composition of the disk. There are two different views on how to treat the chemistry in the midplanes of disks, depending on the scientific focus and heritage. Planet formation and population synthesis models \citep[e.g.,][]{ida2004,ida2008,alibert13} consider multiple physical effects taking place in a protoplanetary disk, such as gravitational interactions between bodies, orbital excitation and eccentricity damping, gas drag, accretion of material onto planets, and planet migration in the gaseous disk. Hence, there is a high degree of physical complexity and detail to planet formation processes in these simulations. However, these models do not contain any detailed chemistry. Either they simply use the observed chemical abundances in interstellar ices and assume that these abundances are preserved during disk evolution, or they assume that thermodynamic equilibrium is attained so that chemical abundances are controlled by temperature and pressure only \citep[e.g.,][]{mousis2010,johnson2012, moses2013,marboeuf14,thiabaud2015gascomp}. The main observational test is through statistical comparisons with the observed populations of exoplanets and their predicted compositions. The alternative view starts from detailed physico-chemical models of protoplanetary disks which are closely linked to, and tested by, a wide variety of astronomical observations (see reviews by \citealt{williams11} and \citealt{armitage2011}). Starting from an assumed (static) surface density distribution, scale height and disk flaring, such models first determine the temperature structure of dust and gas heated by the central star through calculation of the full radiative transfer of the dust and the thermal balance of the gas \citep[e.g.,][]{dullemond2007,nomura2005,woitke2009,bruderer2013}. This physical model is then coupled with an extensive gas-grain chemistry network to solve the kinetic chemistry equations at each point in the disk and compute the chemical composition of the gas and ice as a function of time \citep[e.g.,][]{bergin2007,furuya2014,cleeves2014water,reboussin2015,walsh2012,walsh15}. Since planets are formed in the midplanes of disks, it is particularly important to consider the composition and evolution in the midplanes. To what extent is the initial chemical composition of material that is accreted onto a protoplanetary disk preserved, and what happens to the material after it reaches the midplane of the disk, i.e., to what extent is it reset \citep{visser2009,pontoppidan2014}? Does planetesimal formation happen so fast that ices are incorporated into large bodies early on in the evolution, preventing further chemical processing \citep{zhang2015}? Additional clues to the chemical evolution in disks come from the observations of comets in our own solar system \citep{charnley11}. Cometary records suggest that the chemical composition of the pre-solar nebula has been at least partially preserved in the comet-forming zone throughout its lifetime, pointing to little or no chemical processing. However, the original composition of the material that was present in the protoplanetary disk around the Sun when it formed remains unknown, and studies of other disks are needed to provide a framework for our own solar system. Particularly interesting are the recent results from the ESA {\it Rosetta} mission finding significant amounts of \ce{O2} in comet 67P/C-G \citep[see][]{bieler15}, with similarly high O$_2$ abundances inferred for comet Halley from a re-analysis of the {\it Giotto} data \citep{rubin2015}. Abundances as high as a few~\% of solid \ce{O2} with respect to solid \ce{H2O} are not yet fully understood. Lastly, the deuteration of water and organics also provides insight into the history of the pristine material from the ISM \citep[see][]{ceccarelli2014}. \begin{figure*} \subfigure[][]{\includegraphics[width=0.5\textwidth]{T_N_profile_corrected.pdf}\label{temp_dens}} \subfigure[][]{\includegraphics[width=0.5\textwidth]{cr_level_16.pdf}\label{cr_level}} \caption{(a) The temperature $T(R)$ in K (blue) and number density $n(R)$ in cm$^{-3}$ (red) profiles for the disk midplane. The solid blue line indicates the adjusted temperature profile (as described in the text). The original temperature profile from \citet{alibert13} beyond 5.2~AU is indicated by the dashed blue line. (b) The ionisation rate $\zeta(R)$ in s$^{-1}$ adopted for the disk midplane. The red dashed line depicts the contribution to the ionisation rate from short-lived radionuclides (SLRs) only. The blue dotted line is the contribution from external cosmic rays (CRs) only. The solid green line represents the total ionisation rate (SLRs and CRs) as a function of radial distance.} \label{simple_c} \end{figure*} In their planet population synthesis models, \citet{marboeuf14} assumed the initial chemical abundances to be inherited directly from the interstellar ices observed in dense interstellar clouds. A set of eight volatile molecules (\ce{H2O}, CO, \ce{CO2}, \ce{CH4}, \ce{H2S}, \ce{CH3OH}, \ce{N2}, and \ce{NH3}, species also considered in this work) were homogeneously distributed in their model disk midplane with relative ratios consistent with interstellar ice observations \citep{gibb2004,oberg2011ices,boogert2015}. Depending on the physical conditions in different parts of the midplane, as well as the sublimation temperatures of the species, these molecules could then either be assigned to the gas or ice, with the threshold set by the icelines of the species. Icelines (or snowlines) mark the radius in the disk midplane beyond which species exist solely in ice form and are thus depleted from the gas. This occurs at the radius where the accretion rate onto grain surfaces (or freezeout) exceeds the desorption rate from grain surfaces due to the negative temperature gradient in the midplane. The relative rates of these processes are very strong functions of temperature leading to a narrow transition region from gas to ice (moving outwards in radius). The position of the midplane iceline for a particular species will depend on its volatility (i.e., its binding energy). \citet{marboeuf14} do not consider any chemical reactions in their models, besides freezeout and desorption. The positions of the icelines are important because they determine which species are gas and ice at any location in the disk, and thus which material is available to build larger bodies (solids only). If, for example, a giant planet is forming in the disk, the composition of its core will reflect the ice compositions at the different positions in the disk through which the forming planet has moved. The composition of the planet's atmosphere, on the other hand, will reflect the gas composition at the position where the planet becomes massive enough to accrete an atmosphere onto its surface from the surrounding gas in the disk. Moreover, accretion of icy bodies may still pollute the atmosphere. These pebbles and planetesimals migrate through the disk due to radial drift and may therefore have originated at larger radii. Depending on the pebble and planetesimal sizes, the migration of these objects also affects the location of the icelines \citep[see, e.g.,][]{piso2015}. Particularly important is the C/O ratio of the solid and gaseous material in the disk \citep{oberg2011co}. The ratio depends not only on the different volatilities of the chemical species but also on their production or destruction as a consequence of chemical processing. Since \ce{H2O} and \ce{CO2} (which are both O rich) freeze out at higher temperatures than species that are more C rich, such as \ce{CH4} and CO, the C/O ratio depends on both the physical structure and chemistry in the disk. Ultimately, the chemical composition of a planet's core and its atmosphere may thus differ depending on the history of the disk, the formation location of the planet, and any subsequent migration. To address these questions, we use a physical disk model, in particular its midplane temperature and density, which is the same as that considered in the \citet{marboeuf14} population synthesis models. We compute the abundances of chemical species with time using a comprehensive chemical network and different sets of assumptions (see below) to investigate the degree to which chemical evolution/processing affects the resulting abundances of key volatiles in the disk midplane. The sensitivity of our results to the choice of (i) initial chemical abundances (parent cloud inheritance or chemical reset), (ii) the physical conditions (in particular ionisation level), and (iii) the types of chemical reactions included in the model, are also investigated, with details provided in Sect.~\ref{methods}. This generates eight different simulations, the results of which are presented in Sect.~\ref{results}. Sect.~\ref{discussion} discusses the validity of the inheritance and reset scenarios, the implications for planet formation, and the extent to which the results hold for other disk models. Sect.~\ref{conclusions} summarises the conclusions from this work. \begin{table} \caption{Initial abundances (with respect to H$_{\rm nuc}$) for atomic and molecular initial abundances setups. The binding energies $E_{b}$ for all species are also listed.} % \centering % \begin{tabular}{l l c r} % \hline\hline % Species & Atomic & Molecular &$E_{b}$[K]\\ % \hline % \\ H & 9.1$\times 10^{-5}$ & 5.0$\times 10^{-5}$ & 600\\ % He & 9.8$\times 10^{-2}$ & 9.8$\times 10^{-2}$ & 100\\ \ce{H2} & 5.0$\times 10^{-1}$ & 5.0$\times 10^{-1}$ & 430\\ N & 6.2$\times 10^{-5}$ & & 800\\ O & 5.2$\times 10^{-4}$ & & 800\\ C & 1.8$\times 10^{-4}$ & & 800\\ S & 6.0$\times 10^{-6}$ & & 1100\\ \ce{H2O} & &3.0$\times 10^{-4}$ & 5770\\ CO & &6.0$\times 10^{-5}$ & 855\\ \ce{CO2} & &6.0$\times 10^{-5}$ & 2990\\ \ce{CH4} & &1.8$\times 10^{-5}$ & 1090\\ \ce{N2} & &2.1$\times 10^{-5}$ & 790\\ \ce{NH3} & &2.1$\times 10^{-5}$ & 3130\\ \ce{CH3OH} & &4.5$\times 10^{-5}$ & 4930\\ \ce{H2S} & &6.0$\times 10^{-6}$ & 2743\\ \ce{O2} & &0 & 1000\\ \ce{HCN} & &0 & 3610\\ \ce{NO} & &0 & 1600 \\ \hline % \label{init_abun} \end{tabular} \end{table} | \label{conclusions} The models presented in this work have examined the importance of kinetic chemistry on the molecular composition (gas and ice) in protoplanetary disk midplanes. The main conclusions are listed below. \begin{itemize} \item The disk midplane composition reflects that of interstellar ices only for the case of low ionisation (SLRs only) in the inheritance scenario. The partitioning between gas and ice is determined solely by iceline positions, as is assumed in the planet population synthesis models. The inclusion of grain-surface chemistry has a negligible effect. \item Assuming a higher rate of ionisation (SLRs plus CRs) and inheritance leads to an increase in the abundance of \ce{CH4} ice beyond its iceline, and a significant depletion of gas-phase \ce{CH4} in the critical region between 1 and 15~AU. Cosmic-ray-induced chemistry enables the release of free carbon from CO in the outer disk ($>15$~AU) which is incorporated into gas-phase methane which freezes out. This naturally leads to low gas-phase CO abundances as is observed in some disks. On the other hand, cosmic-ray-induced chemistry efficiently destroys methane gas in the \ce{CH4}-poor region. The conclusion holds whether grain-surface chemistry is included or not. \item When grain-surface chemistry is considered, the \ce{CO2} ice to \ce{H2O} ice ratio is increased, with \ce{CO} and \ce{CH4} gas destroyed at the expense of an increase in \ce{CO2} ice. The critical reactions are the photodissociation of \ce{H2O} ice to form \ce{OH} radicals within the ice mantle, which subsequently react with CO to form \ce{CO2} ice. This reaction is able to proceed faster than H~+~OH recombination within $\approx 20$~AU because the very volatile H atoms are quickly lost to the gas phase for grains warmer than 20~K. Beyond this radius, the reformation of water ice wins. The partitioning between \ce{N2} and \ce{NH3} is similarly affected. \item For the extreme reset scenario in which all elements are initially in atomic form, the picture changes significantly. Without grain-surface chemistry, gas-phase CO, \ce{O2}, and atomic oxygen are the main carbon and oxygen-bearing species beyond 1~AU. The chemistry does not have sufficient time to incorporate all available initial elemental oxygen into molecules by $10^6$~yrs. Gas-phase water and \ce{CO2} do form and subsequently freeze out, albeit achieving much lower abundances than in the inheritance scenario. A higher ionisation level helps to increase the production of \ce{H2O} and \ce{CH4}. \item With grain-surface chemistry, the abundances of \ce{H2O} and \ce{CO2} ice increase significantly, demonstrating the absolute necessity of grain-surface chemistry for the synthesis of these two dominant ice components. The final abundance ratios reached in the very outer disk (30~AU) for \ce{H2O} and \ce{CO2} are similar to those for the inheritance scenario, regardless of the ionisation level; however, the higher ionisation level does impede the abundance of \ce{O2} ice at the levels seen in comets and enables a conversion from CO to \ce{CH4}. \item In the reset scenario, species other than the main considered volatiles are also produced in non-negligible quantities: \ce{O2}, \ce{HCN}, and \ce{NO}. The higher ionisation level generally helps the production of HCN and NO (at the expense of \ce{N2} and \ce{NH3}) and impedes the survival of \ce{O2} ice. \item The inclusion of chemistry has a significant impact on the C/O ratio of both gas and ice in the planet-forming region which is expected to influence the resulting composition of forming planet(esimal)s. The ices remain, on the whole, dominated by oxygen (i.e., C/O $< 1$). For both inheritance cases, the gas is carbon rich relative to the canonical value; however only for the low ionisation case is there a reservoir of gas-phase material with C/O $>1$. For both reset scenarios, the ice becomes more carbon rich than the gas, which is opposite to the inheritance case. \end{itemize} The results presented here show that under certain conditions, highlighted above, chemistry can have a profound effect on the composition of the planet-forming material in disk midplanes. Chemistry influences the partitioning of elemental carbon, oxygen, and nitrogen, into molecules of differing volatilities, such that the positions of ice lines alone, are not necessarily adequate for determining the ratio of C/O in neither the gas, nor the ice. Only under the extreme case of full inheritance and low ionisation, are the elemental ratios determined solely by the positions of icelines. This conclusion is also similar to that for the assumption that ices are already locked up in larger bodies by $\approx 10^{5}$~yr. The work presented here follows the time evolution of chemistry in a static protoplanetary disk which is the simplest physical case. In reality, disk conditions evolve with time, at the same time as planetesimals are forming and migrating within the midplane. Future plans include determining the influence of chemistry in an evolving protoplanetary disk (where the density, temperature, and ionisation rate also vary with time), and to couple the outputs of these models with planet formation tracks to determine, in a quantitative manner, the influence on the resulting composition of gas-giant planetary atmospheres. | 16 | 7 | 1607.06710 | Context. The atmospheres of extrasolar planets are thought to be built largely through accretion of pebbles and planetesimals. Such pebbles are also the building blocks of comets. The chemical composition of their volatiles are usually taken to be inherited from the ices in the collapsing cloud. However, chemistry in the protoplanetary disk midplane can modify the composition of ices and gases. <BR /> Aims: To investigate if and how chemical evolution affects the abundances and distributions of key volatile species in the midplane of a protoplanetary disk in the 0.2-30 AU range. <BR /> Methods: A disk model used in planet population synthesis models is adopted, providing temperature, density and ionisation rate at different radial distances in the disk midplane. A full chemical network including gas-phase, gas-grain interactions and grain-surface chemistry is used to evolve chemistry in time, for 1 Myr. Both molecular (inheritance from the parent cloud) and atomic (chemical reset) initial conditions are investigated. <BR /> Results: Great diversity is observed in the relative abundance ratios of the main considered species: H<SUB>2</SUB>O, CO, CO<SUB>2</SUB>, CH<SUB>4</SUB>, O<SUB>2</SUB>, NH<SUB>3</SUB> and N<SUB>2</SUB>. The choice of ionisation level, the choice of initial abundances, as well as the extent of chemical reaction types included are all factors that affect the chemical evolution. The only exception is the inheritance scenario with a low ionisation level, which results in negligible changes compared with the initial abundances, regardless of whether or not grain-surface chemistry is included. The grain temperature plays an important role, especially in the critical 20-28 K region where atomic H no longer sticks long enough to the surface to react, but atomic O does. Above 28 K, efficient grain-surface production of CO<SUB>2</SUB> ice is seen, as well as O<SUB>2</SUB> gas and ice under certain conditions, at the expense of H<SUB>2</SUB>O and CO. H<SUB>2</SUB>O ice is produced on grain surfaces only below 28 K. For high ionisation levels at intermediate disk radii, CH<SUB>4</SUB> gas is destroyed and converted into CO and CO<SUB>2</SUB> (in contrast with previous models), and similarly NH<SUB>3</SUB> gas is converted into N<SUB>2</SUB>. At large radii around 30 AU, CH<SUB>4</SUB> ice is enhanced leading to a low gaseous CO abundance. As a result, the overall C/O ratios for gas and ice change significantly with radius and with model assumptions. For high ionisation levels, chemical processing becomes significant after a few times 10<SUP>5</SUP> yr. <BR /> Conclusions: Chemistry in the disk midplane needs to be considered in the determination of the volatile composition of planetesimals. In the inner <30 AU disk, interstellar ice abundances are preserved only if the ionisation level is low, or if these species are included in larger bodies within 10<SUP>5</SUP> yr. | false | [
"SUB>2</SUB",
"interstellar ice abundances",
"grain surfaces",
"CO",
"initial abundances",
"ices",
"atomic H",
"intermediate disk radii",
"ionisation level",
"high ionisation levels",
"CO<SUB>2</SUB>",
"gases",
"key volatile species",
"CO. H<SUB>2</SUB>O ice",
"chemical evolution",
"previous models",
"planet population synthesis models",
"a low gaseous CO abundance",
"O<SUB>2</SUB",
"H<SUB>2</SUB"
] | 9.99878 | 13.158843 | -1 |
2774767 | [
"Law, David R.",
"Cherinka, Brian",
"Yan, Renbin",
"Andrews, Brett H.",
"Bershady, Matthew A.",
"Bizyaev, Dmitry",
"Blanc, Guillermo A.",
"Blanton, Michael R.",
"Bolton, Adam S.",
"Brownstein, Joel R.",
"Bundy, Kevin",
"Chen, Yanmei",
"Drory, Niv",
"D'Souza, Richard",
"Fu, Hai",
"Jones, Amy",
"Kauffmann, Guinevere",
"MacDonald, Nicholas",
"Masters, Karen L.",
"Newman, Jeffrey A.",
"Parejko, John K.",
"Sánchez-Gallego, José R.",
"Sánchez, Sebastian F.",
"Schlegel, David J.",
"Thomas, Daniel",
"Wake, David A.",
"Weijmans, Anne-Marie",
"Westfall, Kyle B.",
"Zhang, Kai"
] | 2016AJ....152...83L | [
"The Data Reduction Pipeline for the SDSS-IV MaNGA IFU Galaxy Survey"
] | 359 | [
"Space Telescope Science Institute, 3700 San Martin Drive, Baltimore, MD 21218, USA",
"Center for Astrophysical Sciences, Department of Physics and Astronomy, Johns Hopkins University, Baltimore, MD 21218, USA",
"Department of Physics and Astronomy, University of Kentucky, 505 Rose Street, Lexington, KY 40506-0055, USA",
"Department of Physics and Astronomy and PITT PACC, University of Pittsburgh, 3941 O'Hara Street, Pittsburgh, PA 15260, USA",
"Department of Astronomy, University of Wisconsin-Madison, 475 N. Charter Street, Madison, WI 53706, USA",
"Apache Point Observatory, P.O. Box 59, Sunspot, NM 88349, USA",
"Departamento de Astronomía, Universidad de Chile, Camino del Observatorio 1515, Las Condes, Santiago, Chile ; Centro de Astrofísica y Tecnologías Afines (CATA), Camino del Observatorio 1515, Las Condes, Santiago, Chile ; Visiting Astronomer, Observatories of the Carnegie Institution for Science, 813 Santa Barbara Street, Pasadena, CA, 91101, USA",
"Center for Cosmology and Particle Physics, Department of Physics, New York University, 4 Washington Place, New York, NY 10003, USA",
"Department of Physics and Astronomy, University of Utah, 115 S 1400 E, Salt Lake City, UT 84112, USA",
"Department of Physics and Astronomy, University of Utah, 115 S 1400 E, Salt Lake City, UT 84112, USA",
"Kavli Institute for the Physics and Mathematics of the universe, Todai Institutes for Advanced Study, the University of Tokyo, Kashiwa, 277-8583 (Kavli IPMU, WPI), Japan",
"School of Astronomy and Space Science, Nanjing University, Nanjing 210093, China ; Key Laboratory of Modern Astronomy and Astrophysics (Nanjing University), Ministry of Education, Nanjing 210093, China",
"McDonald Observatory, Department of Astronomy, University of Texas at Austin, 1 University Station, Austin, TX 78712-0259, USA",
"Max Planck Institute for Astrophysics, Karl-Schwarzschild-Str. 1, D-85748 Garching, Germany",
"Department of Physics & Astronomy, University of Iowa, Iowa City, IA 52242, USA",
"Max Planck Institute for Astrophysics, Karl-Schwarzschild-Str. 1, D-85748 Garching, Germany",
"Max Planck Institute for Astrophysics, Karl-Schwarzschild-Str. 1, D-85748 Garching, Germany",
"Department of Astronomy, Box 351580, University of Washington, Seattle, WA 98195, USA",
"Institute of Cosmology and Gravitation, University of Portsmouth, Portsmouth, UK",
"Department of Physics and Astronomy and PITT PACC, University of Pittsburgh, 3941 O'Hara Street, Pittsburgh, PA 15260, USA",
"Department of Astronomy, Box 351580, University of Washington, Seattle, WA 98195, USA",
"Department of Physics and Astronomy, University of Kentucky, 505 Rose Street, Lexington, KY 40506-0055, USA",
"Instituto de Astronomia, Universidad Nacional Autonoma de Mexico, A.P. 70-264, 04510 Mexico D.F., Mexico",
"Physics Division, Lawrence Berkeley National Laboratory, Berkeley, CA 94720-8160, USA",
"Institute of Cosmology and Gravitation, University of Portsmouth, Portsmouth, UK",
"Department of Astronomy, University of Wisconsin-Madison, 475 N. Charter Street, Madison, WI 53706, USA; Department of Physical Sciences, The Open University, Milton Keynes, UK",
"School of Physics and Astronomy, University of St. Andrews, North Haugh, St. Andrews KY16 9SS, UK",
"Institute of Cosmology and Gravitation, University of Portsmouth, Portsmouth, UK",
"Department of Physics and Astronomy, University of Kentucky, 505 Rose Street, Lexington, KY 40506-0055, USA"
] | [
"2016AJ....152..197Y",
"2016MNRAS.463.2513B",
"2017AJ....154...28B",
"2017ApJ...837...32L",
"2017ApJ...838...77L",
"2017ApJ...839...87B",
"2017ApJ...844...80B",
"2017ApJ...850..136S",
"2017ApJ...851...18L",
"2017ApJ...851L..33G",
"2017ApJS..233...25A",
"2017MNRAS.464L..46S",
"2017MNRAS.465..688G",
"2017MNRAS.465.2317J",
"2017MNRAS.465.4572Z",
"2017MNRAS.466.2570B",
"2017MNRAS.466.3217Z",
"2017MNRAS.466.4731G",
"2017MNRAS.467.2612W",
"2017MNRAS.468.4494Z",
"2017MNRAS.469..151B",
"2017MNRAS.470L..49E",
"2017MNRAS.472.4382R",
"2018A&C....25..195F",
"2018ApJ...852...36G",
"2018ApJ...852...74B",
"2018ApJ...854..152A",
"2018ApJ...854..159P",
"2018ApJ...856...93F",
"2018ApJ...856..137W",
"2018ApJ...859....8L",
"2018ApJ...859...37G",
"2018ApJ...863..107D",
"2018ApJ...866..119L",
"2018ApJ...869..117R",
"2018ApJS..235...42A",
"2018JCAP...11..015F",
"2018MNRAS.473.1956I",
"2018MNRAS.473.2160N",
"2018MNRAS.473.2679S",
"2018MNRAS.474.1499W",
"2018MNRAS.474.2323S",
"2018MNRAS.475..716G",
"2018MNRAS.476..580S",
"2018MNRAS.476..979P",
"2018MNRAS.476.1765L",
"2018MNRAS.476.3883L",
"2018MNRAS.477..195T",
"2018MNRAS.477..230R",
"2018MNRAS.477.1567L",
"2018MNRAS.477.3014B",
"2018MNRAS.477.3954P",
"2018MNRAS.477.4711G",
"2018MNRAS.478.5491M",
"2018MNRAS.480.1705L",
"2018MNRAS.480.2217S",
"2018MNRAS.480.2544R",
"2018MNRAS.481.5580F",
"2018RMxAA..54..217S",
"2018arXiv181013242G",
"2019A&A...623A.171P",
"2019AJ....158...74C",
"2019AJ....158..160B",
"2019AJ....158..231W",
"2019ApJ...870...19G",
"2019ApJ...872...63L",
"2019ApJ...872..144H",
"2019ApJ...873...63Z",
"2019ApJ...876...52K",
"2019ApJ...877..132W",
"2019ApJ...880..111O",
"2019ApJ...881..119P",
"2019ApJ...882....9S",
"2019ApJ...882..145B",
"2019ApJ...883...82P",
"2019ApJ...883...83P",
"2019ApJ...883..175Y",
"2019ApJ...883L..36H",
"2019ApJ...884..156S",
"2019ApJS..240...23A",
"2019MNRAS.482..194B",
"2019MNRAS.482.1557S",
"2019MNRAS.482.1733G",
"2019MNRAS.483..172D",
"2019MNRAS.483.2057F",
"2019MNRAS.483.3420P",
"2019MNRAS.483.4525I",
"2019MNRAS.484..252I",
"2019MNRAS.485..428B",
"2019MNRAS.485.1546T",
"2019MNRAS.485.3409G",
"2019MNRAS.485.5256Z",
"2019MNRAS.485.5590R",
"2019MNRAS.486..344R",
"2019MNRAS.486.4463Y",
"2019MNRAS.486.5075D",
"2019MNRAS.487.1927W",
"2019MNRAS.488.3929C",
"2019MNRAS.488L...6F",
"2019MNRAS.489..855C",
"2019MNRAS.489.1338P",
"2019MNRAS.489.1436L",
"2019MNRAS.489.4721V",
"2019MNRAS.489.5709C",
"2019MNRAS.490.2124L",
"2019MNRAS.490.2347Q",
"2019MNRAS.490.3830B",
"2019NatAs...3..178P",
"2019RAA....19...81C",
"2019RAA....19..121Z",
"2019RAA....19..171D",
"2019arXiv190105616Z",
"2019arXiv191005139G",
"2019arXiv191106103G",
"2020A&A...634A.107Y",
"2020A&A...635A..90B",
"2020A&A...636A..42M",
"2020A&A...641A..60P",
"2020A&A...644A.117L",
"2020AJ....159...22L",
"2020ASPC..527..743C",
"2020ApJ...888...88L",
"2020ApJ...890L...3S",
"2020ApJ...892...87W",
"2020ApJ...892L..20F",
"2020ApJ...893...94T",
"2020ApJ...894....3O",
"2020ApJ...894...32G",
"2020ApJ...894...57S",
"2020ApJ...895...25W",
"2020ApJ...895..146C",
"2020ApJ...896...38L",
"2020ApJ...896..121L",
"2020ApJ...898..116K",
"2020ApJ...899...62C",
"2020ApJ...900..109A",
"2020ApJ...901..101S",
"2020ApJ...901..159C",
"2020ApJ...903...16P",
"2020ApJ...903...52S",
"2020ApJ...903..145L",
"2020ApJS..249....3A",
"2020ApJS..249....8B",
"2020ApJS..251...11M",
"2020MNRAS.491.1690J",
"2020MNRAS.491.3655G",
"2020MNRAS.491.3672B",
"2020MNRAS.492...96B",
"2020MNRAS.492.1869D",
"2020MNRAS.492.4680W",
"2020MNRAS.493.3081R",
"2020MNRAS.494.4751M",
"2020MNRAS.495.1958W",
"2020MNRAS.495.2305G",
"2020MNRAS.495.2894D",
"2020MNRAS.495.3387P",
"2020MNRAS.496.1262J",
"2020MNRAS.497.2018H",
"2020MNRAS.497.3011L",
"2020MNRAS.497.4753Z",
"2020MNRAS.498.1002D",
"2020MNRAS.498.1259Z",
"2020MNRAS.498.3985Y",
"2020MNRAS.498.4205V",
"2020MNRAS.498.4550W",
"2020MNRAS.498L..66B",
"2020MNRAS.499..230B",
"2020MNRAS.499.1116F",
"2020MNRAS.499.1406L",
"2020MNRAS.499.5749J",
"2020arXiv201105444O",
"2021A&A...647A.181O",
"2021AJ....161...52L",
"2021ApJ...908..165P",
"2021ApJ...908..183L",
"2021ApJ...909..125X",
"2021ApJ...909..131B",
"2021ApJ...910..162G",
"2021ApJ...911..145Y",
"2021ApJ...912...45N",
"2021ApJ...913...33R",
"2021ApJ...913...44W",
"2021ApJ...915...35L",
"2021ApJ...916...38Z",
"2021ApJ...916..102A",
"2021ApJ...917...72L",
"2021ApJ...919..145R",
"2021ApJ...920...62N",
"2021ApJ...921..177S",
"2021ApJ...922..230R",
"2021ApJ...922..249Y",
"2021ApJ...923...28F",
"2021ApJ...923..120L",
"2021ApJS..254...15A",
"2021ApJS..257...66C",
"2021MNRAS.500L..42P",
"2021MNRAS.501...14L",
"2021MNRAS.501..948B",
"2021MNRAS.501.4064R",
"2021MNRAS.502.3128P",
"2021MNRAS.502L..95G",
"2021MNRAS.503.1345S",
"2021MNRAS.503.5134A",
"2021MNRAS.504.2658Y",
"2021MNRAS.504.4626K",
"2021MNRAS.505..191B",
"2021MNRAS.505.2087K",
"2021MNRAS.505.2447P",
"2021MNRAS.506..727K",
"2021MNRAS.506.4979K",
"2021MNRAS.507.2468S",
"2021MNRAS.507.3923M",
"2021MNRAS.508.1084K",
"2021MNRAS.508.3943J",
"2021MNRAS.508.4844N",
"2021PASJ...73.1152J",
"2021RAA....21..204C",
"2021RAA....21..306Z",
"2021arXiv210208240P",
"2022A&A...659A..79G",
"2022A&A...659A.112J",
"2022A&A...659A.131D",
"2022A&A...660A.145O",
"2022A&A...665A.144M",
"2022A&A...667A..88J",
"2022A&C....4100633D",
"2022AJ....163...56I",
"2022AJ....163...71T",
"2022AJ....164...94B",
"2022AJ....164..127C",
"2022AN....34320048Z",
"2022ApJ...924...47P",
"2022ApJ...925..168Y",
"2022ApJ...926...57Y",
"2022ApJ...926..139M",
"2022ApJ...926L..13B",
"2022ApJ...927...23C",
"2022ApJ...928...58L",
"2022ApJ...929...50P",
"2022ApJ...929..112K",
"2022ApJ...930..160S",
"2022ApJ...932..103G",
"2022ApJ...933...44C",
"2022ApJ...933...61H",
"2022ApJ...933...88O",
"2022ApJ...934..173S",
"2022ApJ...938...96J",
"2022ApJ...940...61F",
"2022ApJS..259...35A",
"2022ApJS..262...36S",
"2022MNRAS.509.4024D",
"2022MNRAS.509.4308H",
"2022MNRAS.510.3119W",
"2022MNRAS.510.3622B",
"2022MNRAS.510.5676I",
"2022MNRAS.511..139B",
"2022MNRAS.511..371E",
"2022MNRAS.511.1913B",
"2022MNRAS.511.4223A",
"2022MNRAS.511.4685X",
"2022MNRAS.513..807D",
"2022MNRAS.513.2904T",
"2022MNRAS.513.5446Z",
"2022MNRAS.513.5988N",
"2022MNRAS.514.2298B",
"2022MNRAS.514.2821B",
"2022MNRAS.514.6120J",
"2022MNRAS.515..320N",
"2022MNRAS.515.1598B",
"2022MNRAS.515.4953T",
"2022MNRAS.515.5081Z",
"2022MNRAS.516.1275G",
"2022MNRAS.516.1442I",
"2022MNRAS.516.3092B",
"2022MNRAS.516.3175B",
"2022MNRAS.517.3723Z",
"2022MNRAS.517.4275H",
"2022MNRAS.517.5660G",
"2023A&A...669A..88P",
"2023A&A...670A.125J",
"2023A&A...673A..12Z",
"2023A&A...673A..23S",
"2023A&A...674A..85A",
"2023A&A...675A.161G",
"2023A&A...678A..65C",
"2023AJ....166...45L",
"2023ApJ...942...25F",
"2023ApJ...945..127N",
"2023ApJ...947...13O",
"2023ApJ...950L..22W",
"2023ApJ...951...52D",
"2023ApJ...952...45B",
"2023ApJ...953...26L",
"2023ApJ...954..115K",
"2023ApJ...957...75Z",
"2023ApJ...958..147P",
"2023AstL...49..151P",
"2023ChPhB..32c9801L",
"2023MNRAS.518.3494B",
"2023MNRAS.518.4713B",
"2023MNRAS.519.1149B",
"2023MNRAS.520.3895G",
"2023MNRAS.520.4301B",
"2023MNRAS.520.5651C",
"2023MNRAS.521.1264T",
"2023MNRAS.521.1775G",
"2023MNRAS.521.4173B",
"2023MNRAS.521.5810Z",
"2023MNRAS.522.1208A",
"2023MNRAS.522.5479N",
"2023MNRAS.522.6326Z",
"2023MNRAS.523..720L",
"2023MNRAS.523.4164H",
"2023MNRAS.523.4251A",
"2023MNRAS.524.5640R",
"2023MNRAS.524.5827F",
"2023MNRAS.525.1008R",
"2023MNRAS.526..904D",
"2023MNRAS.526.1022L",
"2023MNRAS.526.1573S",
"2023NatAs...7..771C",
"2023NatAs...7..951L",
"2023PASA...40....2L",
"2023RAA....23h5001Z",
"2023RMxAA..59..213B",
"2024A&A...681A..93F",
"2024A&A...681A.121S",
"2024A&A...682L..11S",
"2024A&A...684A..32J",
"2024ApJ...961..192W",
"2024ApJ...961..216C",
"2024ApJ...962...27K",
"2024ApJ...962...51J",
"2024ApJ...965..158Y",
"2024ApJ...966..130R",
"2024MNRAS.527..150K",
"2024MNRAS.527..706Z",
"2024MNRAS.527.1580W",
"2024MNRAS.527.1935Z",
"2024MNRAS.527.6419N",
"2024MNRAS.52710788B",
"2024MNRAS.528.2391C",
"2024MNRAS.528.2643B",
"2024MNRAS.528.3559T",
"2024MNRAS.528.4029L",
"2024MNRAS.528.5377C",
"2024MNRAS.529.4500M",
"2024MNRAS.529.4993L",
"2024MNRAS.530.3924E",
"2024MNRAS.530.4082Z",
"2024MNRAS.530.4474L",
"2024MNRAS.531.1592B",
"2024PASJ...76....1T",
"2024RAA....24b5008J",
"2024RASTI...3..234R",
"2024arXiv240108769L",
"2024arXiv240501637D",
"2024arXiv240505960G",
"2024arXiv240512873N"
] | [
"astronomy"
] | 32 | [
"methods: data analysis",
"surveys",
"techniques: imaging spectroscopy",
"Astrophysics - Instrumentation and Methods for Astrophysics"
] | [
"1969PASP...81..527W",
"1982PASP...94..715F",
"1986PASP...98..609H",
"1996PASP..108..277O",
"1998ApJ...500..525S",
"1999ApJ...527L..13C",
"2000A&A...354.1134R",
"2000AJ....120.1579Y",
"2000PASP..112..566M",
"2000SPIE.4008..467B",
"2001MNRAS.326...23B",
"2002MNRAS.329..513D",
"2002PASP..114..144F",
"2003AJ....126.2081A",
"2003MNRAS.341...33K",
"2004ApJ...613..898T",
"2004SPIE.5492.1403K",
"2005PASP..117.1271Z",
"2006A&A...446..747G",
"2006AJ....131.2332G",
"2006AN....327..850S",
"2006ApJS..166..505A",
"2007MNRAS.375.1099D",
"2007NJPh....9..443B",
"2007astro.ph..1297M",
"2008SPIE.7010E..11C",
"2009ApJ...697.2057L",
"2009ApJ...703..785D",
"2009ApJ...706.1364F",
"2009arXiv0910.0167B",
"2010A&A...515A..35S",
"2010Ap&SS.327..245D",
"2010ApJ...716..198B",
"2010MNRAS.404.1775T",
"2010SPIE.7735E..1CW",
"2011AJ....142...31B",
"2011ApJ...739...45F",
"2011ApJ...739L..47B",
"2011ApJS..192....5A",
"2011MNRAS.413..813C",
"2011MNRAS.416.1680C",
"2012A&A...538A...8S",
"2012AJ....144..144B",
"2012MNRAS.421..872C",
"2012Natur.487..338L",
"2012SPIE.8451E..0BW",
"2013A&A...549A..87H",
"2013A&A...557A.130M",
"2013A&A...558A..56D",
"2013AJ....145...10D",
"2013AJ....145..138B",
"2013AJ....146...32S",
"2013ApJS..208....5N",
"2013MNRAS.430.2047H",
"2014A&A...567A..25N",
"2014A&A...569A...1W",
"2014ASPC..485..447S",
"2014ApJ...787...38F",
"2014SPIE.9143E..08B",
"2014SPIE.9147E..24M",
"2014SPIE.9147E..9SW",
"2015A&A...576A.135G",
"2015AJ....149...77D",
"2015AJ....150...19L",
"2015ApJ...798....7B",
"2015ApJ...799..209W",
"2015ApJ...804..125L",
"2015MNRAS.446.1551S",
"2015MNRAS.446.1567A",
"2015MNRAS.449..328W",
"2015MNRAS.449..867B",
"2015PASP..127..646W",
"2016AJ....151....8Y",
"2016AJ....151...44D",
"2016AJ....152..197Y"
] | [
"10.3847/0004-6256/152/4/83",
"10.48550/arXiv.1607.08619"
] | 1607 | 1607.08619.txt | Over the last twenty years, multiplexed spectroscopic surveys have been valuable tools for bringing the power of statistics to bear on the study of galaxy formation. Using large samples of tens to hundreds of thousands of galaxies with optical spectroscopy from the Sloan Digital Sky Survey \citep{york00,dr1.ref} for instance, studies have outlined fundamental relations between stellar mass, metallicity, element abundance ratios, and star formation history \citep[e.g.,][]{kauffmann03,tremonti04,thomas10}. However, this statistical power has historically come at the cost of treating galaxies as point sources, with only a small and biased region subtended by a given optical fiber contributing to the recorded spectrum. % Realizing limitations of such work though; move to IFU spectroscopy. Other major IFU works. As technology has advanced, techniques have been developed for {\it imaging spectroscopy} that allow simultaneous spatial and spectral coverage, with correspondingly greater information density for each individual galaxy. Building on early work by (e.g.) \citet{colina99} and \citet{dezeeuw02}, such integral-field spectroscopy has provided a wealth of information. In the nearby universe for instance, observations from the DiskMass survey \citep{bershady10} have indicated that late-type galaxies tend to have sub-maximal disks \citep{bershady11}, while Atlas-3D observations \citep{cappellari11a} showed that early-type galaxies frequently have rapidly-rotating components \citep[especially in low density environments;][]{cappellari11b}. In the more distant universe, integral-field spectroscopic observations have been crucial in establishing the prevalence of high gas-phase velocity dispersions \citep[e.g.,][]{fs09,law09,law12,wisnioski15}, giant kiloparsec-sized ÒclumpsÓ of young stars \citep[e.g.,][]{fs11}, and powerful nuclear outflows \citep{fs14} that may indicate fundamental differences in gas accretion mechanisms in the young universe \citep[e.g.,][]{dekel09}. More recently, surveys such as CALIFA \citep[Calar Alto Legacy Integral Field Area Survey,][]{sanchez12,califadr2}, SAMI \citep[Sydney-AAO Multi-object IFS,][]{croom12,allen15}, and MaNGA \citep[Mapping Nearby Galaxies at APO,][]{bundy15} have begun to combine the information density of integral field spectroscopy with the statistical power of large multiplexed samples. As a part of the 4th generation of the Sloan Digital Sky Survey (SDSS-IV), the MaNGA project bundles single fibers from the Baryon Oscillation Spectroscopic Survey (BOSS) spectrograph \citep{smee13} into integral-field units (IFUs); over the six-year lifetime of the survey (2014-2020) MaNGA will obtain spatially resolved optical+NIR spectroscopy of 10,000 galaxies at redshifts $z \sim 0.02 - 0.1$. In addition to providing insight into the resolved structure of stellar populations, galactic winds, and dynamical evolution in the local universe \citep[e.g.,][]{belfiore15,li15,wilkinson15}, the MaNGA data set will be an invaluable legacy product with which to help understand galaxies in the distant universe. As next-generation facilities come online in the final years of the MaNGA survey, IFU spectrographs such as TMT/IRIS \citep[][]{moore14,wright14}, JWST/NIRSPEC \citep[][]{closs08,birkmann14}, and JWST/MIRI-MRS \citep[][]{wells15} will trace the crucial rest-optical bandpass in galaxies out to redshift $z \sim 10$ and beyond. % Complications that the MaNGA pipeline must handle. Imaging spectroscopic surveys such as MaNGA face substantial calibration challenges in order to meet the science requirements of the survey \citep{yan16b}. In addition to requiring accurate absolute spectrophotometry from each fiber, MaNGA must correct for gravitationally-induced flexure variability in the Cassegrain-mounted BOSS spectrographs, determine accurate micron-precision astrometry for each IFU bundle, and combine spectra from the individual fibers with accurate astrometric information in order to construct 3-D data cubes that rectify the wavelength-dependent differential atmospheric refraction and (despite large interstitial gaps in the fiber bundles) consistently deliver high-quality {\it imaging} products. These combined requirements have driven a substantial software pipeline development effort throughout the early years of SDSS-IV. % \begin{deluxetable*}{lcccc} \tablecolumns{5} %\tablewidth{100pt} \tabletypesize{\scriptsize} \tablecaption{IFU Data Reduction Software} \tablehead{ \colhead{Telescope} & \colhead{Spectrograph} & \colhead{IFU} & \colhead{Pipeline} & \colhead{Reference}} \startdata \multicolumn{5}{c}{Fiber-Fed IFUs}\\ \hline AAT & AAOMEGA & SAMI & {\sc 2dfdr} & \citet{sharp15}\\ Calar Alto 3.5m & PMAS & PPAK & {\sc p3d} & \citet{sandin10} \\ & & & {\sc r3d} & \citet{sanchez06}\tablenotemark{a}\\ & & & IRAF & \citet{martin13}\tablenotemark{c}\\ HET & VIRUS & VIRUS & {\sc cure} & \citet{snigula14}\\ McDonald 2.7m & VIRUS-P & VIRUS-P &{\sc vaccine} & \citet{adams11}\\ & & & {\sc venga} & \citet{blanc13}\\ SDSS 2.5m & BOSS & MaNGA & {\sc mangadrp} & This paper\\ WHT & WYFFOS & INTEGRAL & {\sc iraf} & \\ WIYN & WIYN Bench Spec. & DensePak & {\sc iraf} & \citet{andersen06}\\ & & SparsePak & {\sc iraf} & \\ \hline \multicolumn{5}{c}{Fiber $+$ Lenslet-Based IFUs}\\ \hline AAT & AAOMEGA & SPIRAL & {\sc 2dfdr} & \citet{hopkins13}\\ Calar Alto 3.5m & PMAS & LARR & As PPAK above & \\ Gemini & GMOS & GMOS & {\sc iraf} & \\ Magellan & IMACS & IMACS & {\sc kungifu} & \citet{bolton07}\\ VLT & GIRAFFE & ARGUS & {\sc girbldrs} & \citet{blecha00} \\ & & & {\sc eso cpl}\tablenotemark{b} & \\ & VIMOS & VIMOS & {\sc vipgi} & \citet{zanichelli05}\\ & & & {\sc eso cpl}\tablenotemark{b} & \\ \hline \multicolumn{5}{c}{Lenslet-Based IFUs}\\ \hline Keck & OSIRIS & OSIRIS & {\sc osirisdrp} & \citet{krabbe04} \\ UH 2.2m & SNIFS & SNIFS & {\sc snurp} & \\ WHT & OASIS & OASIS & {\sc xoasis} & \\ & SAURON & SAURON & {\sc xsauron} & \citet{bacon01}\\ \hline \multicolumn{5}{c}{Slicer-Based IFUs}\\ \hline ANU & WiFeS & WiFeS & {\sc iraf} & \citet{dopita10}\\ Gemini & GNIRS & GNIRS & {\sc iraf} & \\ & NIFS & NIFS & {\sc iraf} & \\ VLT & KMOS & KMOS & {\sc eso cpl}\tablenotemark{b}, {\sc spark} & \citet{davies13}\\ & MUSE & MUSE & {\sc eso cpl}\tablenotemark{b} & \citet{weil12}\\ & SINFONI & SINFONI & {\sc eso cpl}\tablenotemark{b} & \citet{modig07}\\ \enddata \tablenotetext{a}{See \citet{sanchez12} for details of the implementation for the CALIFA survey.} \tablenotetext{b}{See http://www.eso.org/sci/software/cpl/} \tablenotetext{c}{Reference corresponds to the DiskMass survey.} \label{software.table} \end{deluxetable*} Historically, IFU data have been processed with a mixture of software tools ranging from custom built pipelines \citep[e.g.,][]{zanichelli05} to general purpose tools capable of performing all or part of the basic data reduction tasks for multiple IFUs. For fiber-fed IFUs (with or without coupled lenslet arrays) that deliver a pseudo-slit of discrete apertures the raw data is similar in format to traditional multiobject spectroscopy and has hence been able to build upon an existing code base. In contrast, slicer-based IFUs produce data in a format more akin to long-slit spectroscopy, while pure-lenslet IFUs are different altogether with individual spectra staggered across the detector. Following \citet[][]{sandin10}, we provide here a brief overview of some of the common tools for the reduction of data from optical and near-IR IFUs \citep[see also][]{bershady09}, including both fiber-fed IFUs with data formats similar to MaNGA and lenslet- and slicer-based IFUs by way of comparison. As shown in Table \ref{software.table}, the {\sc iraf} environment remains a common framework for the reduction of data from many facilities, especially Gemini, WIYN, and the WHT. Similarly, the various IFUs at the VLT can all be reduced with software from a common ISO C-based pipeline library, although some other packages \citep[e.g., GIRBLDRS,][]{blecha00} are also capable of reducing data from some VLT IFUs. Substantial effort has been invested in the {\sc p3d} \citep{sandin10} and {\sc r3d} \citep{sanchez06} packages as well, which together are capable of reducing data from a wide variety of fiber-fed instruments (including PPAK/LARR, VIRUS-P, SPIRAL, GMOS, VIMOS, INTEGRAL, and SparsePak) for which similar extraction and calibration algorithms are generally possible. For survey-style operations, the SAMI survey has adopted a two-stage approach, combining a general-purpose spectroscopic pipeline {\sc 2dfdr} \citep{hopkins13} with a custom three-dimensional stage to assemble IFU data cubes from individual fiber spectra \citep{sharp15}. Similarly, the MaNGA Data Reduction Pipeline ({\mangadrp}; hereafter the DRP) is also divided into two components. Like the {\sc kungifu} package \citep{bolton07}, the 2d stage of the DRP is based largely on the SDSS BOSS spectroscopic reduction pipeline {\sc idlspec2d} (Schlegel et al., in prep), and processes the raw CCD data to produce sky-subtracted, flux-calibrated spectra for each fiber. The 3d stage of the DRP is custom built for MaNGA, but adapts core algorithms from the CALIFA \citep{sanchez12} and VENGA \citep{blanc13} pipelines in order to produce astrometrically registered composite data cubes. In the present contribution, we describe version v1\_5\_4 of the MaNGA DRP corresponding to the first public release of science data products in SDSS Data-Release 13 (DR13)\footnote{DR13 is available at http://www.sdss.org/dr13/}. We start by providing a brief overview of the MaNGA hardware and operational strategy in \S \ref{hardwareops.sec}, and give an overview of the DRP and related systems in \S \ref{drpoverview.sec}. We then discuss the individual elements of the DRP in detail, starting with the basic spectral extraction technique (including detector preprocessing, fiber tracing, flatfield and wavelength calibration) in \S \ref{drp2d.1}. In \S \ref{skysub.sec} we discuss our method of subtracting the sky background (including the bright atmospheric OH features) from the science spectra, and demonstrate that we achieve nearly Poisson limited performance shortward of 8500 \AA. In \S \ref{fluxcal.sec} we discuss the method for spectrophotometric calibration of the MaNGA spectra, and in \S \ref{waverect.sec} our approach to resampling and combining all of the individual spectra onto a common wavelength solution. We describe the astrometric calibration in \S \ref{astrometry.sec}, combining a basic approach that takes into account fiber bundle metrology, differential atmospheric refraction, and other factors (\S \ref{basicast.sec}) and an `extended' astrometry module that registers the MaNGA spectra against SDSS-I broadband imaging (\S \ref{eam.sec}). Using this astrometric information we combine together individual fiber spectra into composite 3d data cubes in \S \ref{cubes.sec}. Finally, we assess the quality of the MaNGA DR13 data products in \S \ref{dq.sec}, focusing on the effective angular and spectral resolution, wavelength calibration accuracy, and typical depth of the MaNGA spectra compared to other extant surveys. We summarize our conclusions in \S \ref{summary.sec}. Additionally, we provide an Appendix \ref{datamodel.sec} in which we outline the structure of the MaNGA DR13 data products and quality-assessment bitmasks. %%%%%%%%%%%%%%%%%%%%%%%%%%%%%%%%%%%%%%%%% % Hardware Design and Observing %%%%%%%%%%%%%%%%%%%%%%%%%%%%%%%%%%%%%%%%% | \label{summary.sec} The 13th data release of the Sloan Digital Sky Survey includes the raw MaNGA spectroscopic data, the fully reduced spectrophotometrically calibrated data, and the pipeline software and metadata required for individual users to re-reduce the data themselves. In this work, we have described the framework and algorithms of the MaNGA Data Reduction Pipeline software \mangadrp\ version v1\_5\_4 and the format and quality of the ensuing reduced data products. The DRP operates in two stages; the first stage performs optimal extraction, sky subtraction, and flux calibration of individual frames, while the second combines multiple frames together with astrometric information to create calibrated individual fiber spectra (in a row-stacked format) and rectified coadded data cubes for each target galaxy. The row-stacked spectra and coadded data cubes are provided for both a linear and a logarithmically sampled wavelength grid, both covering the wavelength range 3622 - 10,354 \AA. For the 1390 galaxy data cubes released in DR13 we demonstrate that the MaNGA data have nearly Poisson-limited sky subtraction shortward of $\sim$ 8500 \AA, with a residual pixel value distribution in all-sky test plates nearly consistent with a Gaussian distribution whose width is determined by the expected contributions from detector and Poisson noise. Each MaNGA exposure is flux calibrated independently of all other exposures using mini-bundles placed on spectrophotometric standard stars; based on comparison to broadband imaging the composite data cubes have a typical relative calibration of 1.7\% (between \Hb\ and \Ha) with an absolute calibration of better than 5\% for more than 89\% of the MaNGA wavelength range. These data cubes reach a typical $10\sigma$ limiting continuum surface brightness $\mu = 23.5$ AB arcsec$^{-2}$ in a five arcsec diameter aperture in the $g$ band. Additionally, we have demonstrated that: \begin{itemize} \item The wavelength calibration of the MaNGA data has an absolute accuracy of 5 \kms\ rms with a relative fiber-to-fiber accuracy of better than 1 \kms\ rms. \item The astrometric accuracy of the reconstructed MaNGA data cubes is typically 0.1 arcsec rms, based on comparison to previous SDSS broadband imaging. \item The spatial resolution of the MaNGA data is a function of the observational seeing, with a median of 2.54 arcsec FWHM. We have shown that the effective reconstructed point source profile is well described by a single gaussian whose parameters are given in the header of each data cube. \item The spectral resolution of the MaNGA data is a function of both both fiber number and wavelength, but has a median $\sigma = 72$ \kms. \end{itemize} Despite these overall successes of the MaNGA DRP, we conclude by noting that there is still ample room for future improvements to be made in some key areas. First, sky subtraction (while adequate for most purposes) shows some non-gaussianities in the residual distribution, a slight overstimate in the read noise of one camera, and a possible systematic oversubtraction at the $\sim 0.1\sigma$ level in the blue. Work is ongoing to test whether better treatment of amplifier crosstalk or the scattered light model can improve limiting performance in this area for the purposes of extremely deep spectral stacking. Secondly, the spectral line spread functions given in the DR13 data products (and in previous SDSS optical fiber spectra) are effectively under-reported by about 10\%. Work is currently underway to use high spectral resolution observations of MaNGA target galaxies to constrain this effect more precisely and fix it in future data releases. Third, spatial covariance in the reconstructed data cubes (treated here by a simple functional approximation) can also be treated more completely. Finally, with additional data it will be possible to fine tune the MaNGA quality control algorithms (which currently can be overly aggressive in flagging potentially problematic cases) and likely recover some of the objects whose reduced data have been identified as unreliable for use in DR13. | 16 | 7 | 1607.08619 | Mapping Nearby Galaxies at Apache Point Observatory (MaNGA) is an optical fiber-bundle integral-field unit (IFU) spectroscopic survey that is one of three core programs in the fourth-generation Sloan Digital Sky Survey (SDSS-IV). With a spectral coverage of 3622-10354 Å and an average footprint of ∼500 arcsec<SUP>2</SUP> per IFU the scientific data products derived from MaNGA will permit exploration of the internal structure of a statistically large sample of 10,000 low-redshift galaxies in unprecedented detail. Comprising 174 individually pluggable science and calibration IFUs with a near-constant data stream, MaNGA is expected to obtain ∼100 million raw-frame spectra and ∼10 million reduced galaxy spectra over the six-year lifetime of the survey. In this contribution, we describe the MaNGA Data Reduction Pipeline algorithms and centralized metadata framework that produce sky-subtracted spectrophotometrically calibrated spectra and rectified three-dimensional data cubes that combine individual dithered observations. For the 1390 galaxy data cubes released in Summer 2016 as part of SDSS-IV Data Release 13, we demonstrate that the MaNGA data have nearly Poisson-limited sky subtraction shortward of ∼8500 Å and reach a typical 10σ limiting continuum surface brightness μ = 23.5 AB arcsec<SUP>-2</SUP> in a five-arcsecond-diameter aperture in the g-band. The wavelength calibration of the MaNGA data is accurate to 5 km s<SUP>-1</SUP> rms, with a median spatial resolution of 2.54 arcsec FWHM (1.8 kpc at the median redshift of 0.037) and a median spectral resolution of σ = 72 km s<SUP>-1</SUP>. | false | [
"Sloan Digital Sky Survey",
"MaNGA",
"individual dithered observations",
"SDSS-IV Data Release",
"centralized metadata framework",
"unprecedented detail",
"the MaNGA Data Reduction Pipeline algorithms",
"∼8500 Å",
"SDSS-IV Data",
"the MaNGA Data Reduction Pipeline",
"Mapping Nearby Galaxies",
"fourth",
"a median spatial resolution",
"s",
"a median spectral resolution",
"IFU",
"the MaNGA data",
"the fourth-generation Sloan Digital Sky Survey",
"∼500 arcsec",
"limiting continuum surface brightness μ = 23.5 AB arcsec"
] | 11.558747 | 6.792415 | 189 |
12585901 | [
"Couturier, C.",
"Zopounidis, J. P.",
"Sauzet, N.",
"Naraghi, F.",
"Santos, D."
] | 2017JCAP...01..027C | [
"Dark matter directional detection: comparison of the track direction determination"
] | 10 | [
"LPSC, CNRS/IN2P3, UJF, INP, Avenue des Martyrs, 38000, Grenoble, France",
"LPSC, CNRS/IN2P3, UJF, INP, Avenue des Martyrs, 38000, Grenoble, France",
"LPSC, CNRS/IN2P3, UJF, INP, Avenue des Martyrs, 38000, Grenoble, France",
"LPSC, CNRS/IN2P3, UJF, INP, Avenue des Martyrs, 38000, Grenoble, France",
"LPSC, CNRS/IN2P3, UJF, INP, Avenue des Martyrs, 38000, Grenoble, France"
] | [
"2016arXiv160708765C",
"2017JInst..12P0009B",
"2017PhRvD..96h3011O",
"2020PhRvD.102g5036B",
"2020PhRvD.102i1701D",
"2020PhRvD.102l3028P",
"2021JCAP...04..047A",
"2021Univ....7..215A",
"2022PAN....85...97A",
"2022PhRvD.105f3014H"
] | [
"astronomy",
"physics"
] | 3 | [
"Astrophysics - Instrumentation and Methods for Astrophysics",
"Physics - Instrumentation and Detectors"
] | [
"1985PhRvD..31.3059G",
"1988PhRvD..37.1353S",
"2003PhLB..571..132S",
"2010NIMPB.268.1818Z",
"2012EAS....53...11D",
"2012EAS....53...33M",
"2012PhRvD..85c5006B",
"2013EPJC...73.2276C",
"2013JPhCS.469a2001B",
"2013JPhCS.469a2002S",
"2014PhRvD..89b3524B",
"2016NIMPA.832..214M",
"2016PhR...662....1B"
] | [
"10.1088/1475-7516/2017/01/027",
"10.48550/arXiv.1607.08157"
] | 1607 | 1607.08157_arXiv.txt | Weakly interacting massive particles are among the most studied candidates for Dark Matter (DM). The direct search for DM is performed by looking for elastic collisions of these WIMPs with target nuclei in a detector~\cite{goodman_1985}. For a 1\,GeV/c$^2$ to 1000\,GeV/c$^2$ DM particle, the kinetic energy of the recoiling nuclei would be in the range of few keV to few hundreds of keV depending on the mass of the target nuclei. The search for such rare events, at low energies, requires a highly efficient discrimination of the background with respect to signal composed of nuclear recoils. Non-directional detection is mainly limited -- after an ideal electron-recoil discrimination -- by two non-reducible background components: the neutrons and the neutrinos. Neutron background comes from natural radioactivity of the detector itself and the surrounding environment ($^{238}$U and $^{232}$Th) and from cosmic muons interacting with the rock and the detector. This background is present in all detectors, no matter how large the fiducial volume is. A veto can help to partly discriminate this background ; however the neutrons produced in the shielding or inside the detector are hard to discriminate. Neutrinos may come from the Sun, the atmosphere (interaction with cosmic rays) and from the DSNB (Diffuse Supernova Neutrino Background). The coherent scattering of these neutrinos on the target nuclei produces recoils in the energy region of interest (\mbox{E$_R$ $\lesssim$ 100\,keV}). When scaling the experiment, the neutrino background becomes non negligible. This ultimate neutrino background, called ``neutrino floor'', cannot be discriminated with standard non-directional methods, as no veto or shielding can be set for neutrinos and thus enforces a lower limit to the cross section attainable with non-directional detectors~\cite{billard_2014}. On the other hand, directional detection proposes to use the anisotropy in the recoil angular distribution (in the galactic coordinates) originated from the motion of the solar system around the galactic center within the DM halo~\cite{spergel_1988}. The mostly isotropic background can thus be distinguished from the (anisotropic) expected DM signal. In case of a claimed detection by a non-directional detector, a directional detection would be an unambiguous proof of the DM origin of the claimed signal~\cite{billard_2012}. The crucial point of the directional detection is to get access to the initial direction of the recoil nucleus. Several strategies have been proposed for a directional detection. We will focus on three strategies and present them: anisotropic crystals, nuclear emulsions and low pressure gaseous TPCs. The goal here is to provide a method to assess how the information of the initial direction of a nuclear recoil is retained in a given material. The direction actually measured by a detector is mainly defined by the WIMP-ion recoil angular distribution. Instrumental effects might dilute the initial direction information: readout \cite{battat_readout_2016}, diffusion effects. We will avoid the WIMP-ion kinematic effects, and will focus on the motion of the first recoiling nucleus in the detector material, and consider it has a fixed energy and a fixed direction. We use SRIM simulations~\cite{ziegler_2008,ziegler_2010} to emulate the motion of such nuclear recoil in each detector material; the SRIM outputs will help us define a figure of merit evaluating the preservation of the direction of a nuclear recoil in each material. | \label{sec:discussion} Using SRIM simulations, we compared how the recoil primary direction information is preserved in the detector materials for three different strategies for a Dark matter directional detection: anisotropic crystals, emulsion layers and low-pressure gaseous TPCs. Anisotropic crystals do not allow an event by event reconstruction of the tracks; yet showing how they preserve the direction information is useful to understand the prospective results of a directional measurement. On the other hand, emulsion and TPCs detectors measure each recoil track; the ranges of the typical recoil tracks expected from the elastic scattering by a WIMP is consistent with the reconstruction resolution of their respective readouts. We propose the use of a new observable, \textit{D}, to quantify the preservation of the initial direction information of the recoiling nucleus. This observable shows that among the three studied materials, a low pressure TPC gaseous mix is better suited for measuring the direction of WIMP-induced nuclear recoils. In fact, dedicated measurements with a calibration source producing ions with a known direction at a given kinetic energy such as the COMIMAC facility \cite{muraz_comimac_2016} would allow to confirm the simulations presented here. \vspace{5mm} We acknowledge F. Hosseini and Q. Riffard help during the phase of preliminary data analysis. CC acknowdleges financial support from the Labex Enigmass. | 16 | 7 | 1607.08157 | Several directional techniques have been proposed for a directional detection of Dark matter, among others anisotropic crystal detectors, nuclear emulsion plates, and low-pressure gaseous TPCs. The key point is to get access to the initial direction of the nucleus recoiling due to the elastic scattering by a WIMP. In this article, we aim at estimating, for each method, how the information of the recoil track initial direction is preserved in different detector materials. We use the SRIM simulation code to emulate the motion of the first recoiling nucleus in each material. We propose the use of a new observable, D, to quantify the preservation of the initial direction of the recoiling nucleus in the detector. We show that in an emulsion mix and an anisotropic crystal, the initial direction is lost very early, while in a typical TPC gas mix, the direction is well preserved. | false | [
"initial direction",
"different detector materials",
"crystal detectors",
"nuclear emulsion plates",
"WIMP",
"low-pressure gaseous TPCs",
"the initial direction",
"the first recoiling nucleus",
"a typical TPC gas mix",
"the recoiling nucleus",
"Dark matter",
"TPC",
"Several directional techniques",
"first",
"others",
"an emulsion mix",
"the direction",
"the detector",
"the elastic scattering",
"an anisotropic crystal"
] | 7.508951 | -2.481737 | 54 |
12509827 | [
"Obata, Ippei",
"Soda, Jiro"
] | 2016PhRvD..94d4062O | [
"Oscillating chiral tensor spectrum from axionic inflation"
] | 42 | [
"Department of Physics, Kyoto University, Kyoto 606-8502, Japan",
"Department of Physics, Kobe University, Kobe 657-8501, Japan"
] | [
"2016JCAP...12..031G",
"2016JHEP...12..137A",
"2017JCAP...06..050O",
"2017JCAP...08..005G",
"2017JHEP...03..024C",
"2017JHEP...08..130A",
"2017PhRvD..95f3533J",
"2017PhRvD..95h3519P",
"2017PhRvD..96b3501A",
"2017arXiv170201193B",
"2018JCAP...07..023F",
"2018JCAP...09..030P",
"2018JHEP...10..193A",
"2018arXiv181007188A",
"2019JCAP...03..032T",
"2019JCAP...04..044F",
"2019JCAP...05..057F",
"2019JCAP...07..004P",
"2019PhRvD..99b3513O",
"2020EL....12940001O",
"2020EPJC...80..145B",
"2020JCAP...05..007K",
"2020JCAP...11..009B",
"2020PDU....2800514N",
"2020PhLB..80135136A",
"2020PhRvD.101d4009O",
"2020PhRvD.101f4057G",
"2020PhRvD.102l3527N",
"2020Symm...12..672K",
"2021JCAP...03..047H",
"2021JCAP...03..073B",
"2021PhRvD.103d4036O",
"2022EL....13969004O",
"2022JCAP...01..007F",
"2022PhRvD.105j3516T",
"2022PhRvD.105j3518F",
"2022PhRvD.106d4041O",
"2023JHEP...01..099B",
"2023PDU....4001186O",
"2023arXiv230702322U",
"2024arXiv240405464A",
"2024arXiv240611742B"
] | [
"astronomy",
"physics"
] | 6 | [
"Astrophysics - Cosmology and Nongalactic Astrophysics",
"General Relativity and Quantum Cosmology",
"High Energy Physics - Phenomenology",
"High Energy Physics - Theory"
] | [
"1990PhRvL..65.3233F",
"1998PhRvD..57.7089C",
"2000PhR...331..283M",
"2001PhRvL..87v1103S",
"2005IJMPD..14.1347W",
"2005JCAP...01..005K",
"2005PhRvD..72h3005C",
"2006PhRvD..73b3504S",
"2006PhRvD..73f3001S",
"2008JCAP...04..010C",
"2008PhRvD..78j6003S",
"2010JCAP...06..009F",
"2010PhRvD..82d6003M",
"2011JCAP...01..017F",
"2011JCAP...01..026K",
"2011JCAP...04..009B",
"2011JCAP...06..003S",
"2011PhRvD..83d4011Y",
"2012PhRvD..85b3534C",
"2012PhRvD..86f9901C",
"2012PhRvD..86j3508B",
"2012PhRvL.108z1302A",
"2013JCAP...09..018P",
"2013JHEP...09..087A",
"2013PhRvD..87h3526A",
"2013PhRvD..87j3501D",
"2013PhRvD..88b1302A",
"2014JCAP...02..037E",
"2014JHEP...09..123M",
"2014PTEP.2014j3C01K",
"2014PhRvD..89f3536M",
"2014PhRvD..89f3537M",
"2015PhRvD..91j3001C",
"2015PhRvD..92f3516O",
"2015PhRvL.114o1303D",
"2016A&A...594A..17P",
"2016JCAP...01..041N",
"2016JCAP...06..031D",
"2016JHEP...02..059C",
"2016PhLB..753..653K",
"2016PhLB..759..520C",
"2016PhRvD..93f3005C",
"2016PhRvD..93j1302P",
"2016PhRvD..93l3502O",
"2017JCAP...10..055F"
] | [
"10.1103/PhysRevD.94.044062",
"10.48550/arXiv.1607.01847"
] | 1607 | 1607.01847_arXiv.txt | Inflation can be regarded as magnifier which allows us to probe microscopic world, namely, high energy physics through the cosmic microwave background (CMB) anisotropies or the large scale structures of the universe. Indeed, it is important to explore high energy physics such as string theory by means of inflation. It is known that there are a lot of scalar fields called axions and gauge fields in string theory. It should be noted that an axion is one of the best-motivated candidates of an inflaton since it naturally gives rise to a nearly flat potential protected by its shift symmetry \cite{Freese:1990rb}. One of the characteristic feature of axionic inflation is that it gets correction of the form of the periodic potential due to quantum non-perturbative effects such as instantons. Specific examples are the axion monodromy mechanism \cite{Silverstein:2008sg} or a kind of aligned natural inflation motivated by the weak gravity conjecture \cite{Kim:2004rp, delaFuente:2014aca}. Remarkably, this kind of potential gives the small modulation to the scalar power spectrum. Thus, the oscillatory feature in the power-spectrum is intimately related to fundamental physics. Intriguingly, although the oscillation in the scalar spectrum has been often discussed~\cite{Wang:2002hf, Easther:2013kla}, the oscillation in the tensor spectrum has been overlooked. This is because, on the CMB scales, the oscillation amplitude in the tensor spectrum is suppressed by several orders of magnitude compared to that in scalar spectrum. However, it is worth seeking the possibility of this oscillatory signature in the tensor spectrum on the scales probed by future space-based gravitational wave experiments such as DECIGO \cite{Kawamura:2011zz} and BBO \cite{Crowder:2005nr}. In this work, we explore the possibility of generating primordial gravitational waves (PGWs) with oscillatory features in axionic inflation. Specifically, we focus on two types of axionic inflations: one is single-field axion monodromy inflation and the other is a variant of inflation driven by the axion coupled to SU(2) gauge field, called aligned chromo-natural inflation \cite{Adshead:2012kp}. These two models are quite different from the point of the mechanism of producing tensor spectra. In the case of single-field monodromy inflation, the tensor spectrum comes from vacuum fluctuations. We see that it is difficult to detect the oscillatory feature from single-field monodromy inflation since the amplitude of oscillation is suppressed by the factor of slow-roll parameters and sub-Planckian decay constant. On the other hand, in the case of aligned chromo-natural inflation, the tachyonic growth of one helicity mode of the gauge field produces chiral PGWs during inflation \cite{Dimastrogiovanni:2012ew, Adshead:2013qp, Obata:2014loa, Obata:2016tmo}. We find that the tensor mode due to particle production of gauge field is sensitive to the modulation of inflaton potential and it produces detectable oscillatory feature in the tensor spectrum even for the tiny modulation. This feature will open up a new window to physics in early universe through the chiral gravitational waves. | We studied the oscillatory feature of tensor spectrum from axionic inflation. In the case of single-field monodromy inflation, the modulation in tensor spectrum is too small to be detected by DECIGO or BBO. On the other hand, in the case of aligned chromo-natural inflation, we can get the sizable modulation in one helicity mode of tensor perturbation sourced by the gauge field which experienced the tachyonic instability around horizon crossing during inflation. Thus, we found the possibility of producing sizable oscillatory feature in the tensor spectrum of chiral gravitational waves from axionic inflations when axion couples to the gauge field during inflation. In this work, we discussed the tensor spectrum with a sizable oscillation produced by non-Abelian gauge field in chromo-natural inflation. It is known that it is difficult to reconcile the original chromo-natural model with CMB data because it yields too large red scalar spectral index or too much chiral GWs. However, it is possible to improve the model so that chromo-natural inflation occurs in a frequency range higher than nHz and CMB constraints can be satisfied~\cite{Obata:2016tmo}. Moreover, we can expect the sizable modulation in the tensor spectrum in the case of Abelian gauge field because one helicity mode of gauge field produces tensor modes at the non-linear level \cite{Sorbo:2011rz}. We leave these issues for future work. | 16 | 7 | 1607.01847 | We study axionic inflation with a modulated potential and examine if the primordial tensor power spectrum exhibits oscillatory feature, which is testable with future space-based gravitational-wave experiments such as DECIGO and BBO. In the case of single-field axion monodromy inflation, it turns out that it is difficult to detect an oscillation in the spectrum due to the suppression of the sub-Planckian decay constant of the axion. On the other hand, in the case of aligned chromo-natural inflation where the axion is coupled to a SU(2) gauge field, it turns out that a sizable oscillation in the tensor spectrum can occur due to the enhancement of chiral gravitational waves sourced by the gauge field. We expect that this feature will be a new probe for axion phenomenologies in the early Universe through chiral gravitational waves. | false | [
"chiral gravitational waves",
"axion phenomenologies",
"BBO",
"single-field axion monodromy inflation",
"oscillatory feature",
"axionic inflation",
"DECIGO",
"future space-based gravitational-wave experiments",
"the sub-Planckian decay constant",
"the gauge field",
"the tensor spectrum",
"Planckian",
"a sizable oscillation",
"the primordial tensor power",
"a SU(2) gauge field",
"aligned chromo-natural inflation",
"the axion",
"the spectrum",
"the early Universe",
"an oscillation"
] | 10.638771 | -1.320895 | 89 |
2775537 | [
"Park, Geumsook",
"Koo, Bon-Chul",
"Kang, Ji-hyun",
"Gibson, Steven J.",
"Peek, J. E. G.",
"Douglas, Kevin A.",
"Korpela, Eric J.",
"Heiles, Carl E."
] | 2016ApJ...827L..27P | [
"A High-velocity Cloud Impact Forming a Supershell in the Milky Way"
] | 10 | [
"Department of Physics and Astronomy, Seoul National University Seoul 151-747, Korea",
"Department of Physics and Astronomy, Seoul National University Seoul 151-747, Korea",
"Korea Astronomy and Space Science Institute Daejeon 305-348, Korea",
"Department of Physics and Astronomy, Western Kentucky University 1906 College Heights Blvd., Bowling Green, KY 42101, USA",
"Space Telescope Science Institute 3700 San Martin Dr., Baltimore, MD 21218, USA",
"Department of Physics and Astronomy, Okanagan College 1000 K. L. O. Rd., Kelowna, British Columbia V1Y 4X8, Canada",
"Space Sciences Laboratory, University of California Berkeley, CA 94720, USA",
"Radio Astronomy Lab, UC Berkeley 601 Campbell Hall, Berkeley, CA 94720, USA"
] | [
"2017AIPC.1792d0007M",
"2017ApJ...849...22I",
"2018A&A...619A.101E",
"2018ApJ...856..166H",
"2018ApJS..234....2P",
"2018MNRAS.478L..72R",
"2020AJ....160...66P",
"2021A&A...645A.113K",
"2021AJ....161...93D",
"2023ApJ...943...55L"
] | [
"astronomy"
] | 7 | [
"Galaxy: disk",
"ISM: clouds",
"radio lines: ISM",
"Astrophysics - Astrophysics of Galaxies"
] | [
"1979ApJ...229..533H",
"1980A&A....88...61T",
"1984ApJS...55..585H",
"1987A&A...179..219T",
"1990ApJ...356..130M",
"1991A&A...244L..29K",
"1991A&A...250..499W",
"1991A&A...250..509W",
"1997ApJS..109..139T",
"1998PASA...15..147D",
"1999A&A...341..437B",
"1999A&A...349..389V",
"1999AJ....118..323R",
"1999ApJ...515..657S",
"2001A&A...370L..26B",
"2002A&A...391..159D",
"2002AJ....123..873P",
"2002ApJ...578..176M",
"2003ApJS..146..407F",
"2003ApJS..149..405H",
"2004Ap&SS.289..381W",
"2005A&A...432..937W",
"2006ApJ...643..881L",
"2007ApJS..173...85K",
"2009ApJ...698.1485H",
"2009ApJ...699.1775K",
"2010AJ....140..262K",
"2010MNRAS.408L..85J",
"2011A&A...533A.105W",
"2011AJ....141...23B",
"2011ApJ...734L..24D",
"2011ApJS..194...32A",
"2011MNRAS.418.1575P",
"2011piim.book.....D",
"2012A&A...547A..43P",
"2012AAS...21934929G",
"2012ARA&A..50..491P",
"2014A&A...563A..99F",
"2014ApJS..212....1A",
"2016MNRAS.462L..46H"
] | [
"10.3847/2041-8205/827/2/L27",
"10.48550/arXiv.1607.07699"
] | 1607 | 1607.07699_arXiv.txt | \label{sec:intro} Supershells are large gaseous shells of radius greater than a few hundred parsecs. They are distinct from other shell-like structures in their extraordinarily large energy requirement, i.e., $\ga 3\times 10^{52}$~erg, which corresponds to $\ga 30$ supernova (SN) explosions \citep{heiles1979,heiles1984,mcclure2002}. About twenty supershells have been found in the Milky Way, and numerous neutral atomic hydrogen (\schi) holes corresponding to supershells have been discovered in nearby dwarfs and spiral galaxies \citep{kamphuis1991,bagetakos2011}. These gigantic structures are generally thought to arise from multiple SN explosions in stellar OB associations. But most supershells are missing a stellar association in their interior, and the number of supershells and their energies are usually incompatible with the level of star formation in those galaxies \citep{heiles1984,rhode1999}. Therefore, several alternative scenarios have been proposed, the most popular of which is the collision of high-velocity clouds (HVCs) with the disk \citep{tenorio1980,tenorio1987,mirabel1990}. HVCs are \schi\ clouds with radial velocities very different from the disk material in the Milky Way, e.g., with a deviation more than 50~\kms\ from the range of permitted velocities in a simple model of the distribution and rotation of the \schi\ gas in the Galaxy \citep{wakker1991a}. Some large HVC complexes are known to be gas streams tidally stripped from satellite galaxies of the Milky Way, but the origin of isolated compact HVCs (CHVCs) remain controversial: they could be clouds formed from galactic fountain or intergalactic accreting flows, part of the large HVC complexes, or condensations in the multi-phase circumgalactic medium \citep{putman2011, wakker2004, putman2012}. The HVC origin has been proposed for a few supershells \citep{heiles1984, mirabel1990,tamanaha1997}, but there has been no clear example showing a direct link between the two, particularly for CHVCs. Here we report the detection of a Galactic supershell with an associated HVC, GS040.2+00.6$-$70 (hereafter GS040). GS040 was first identified as a faint, forbidden-velocity wing feature (FVW~40.0+0.5) in the low-resolution, large-scale longitude-velocity study of \cite{kang2007}. We have found that GS040 appears to be a complete circular ring with complicated structures inside in our high-resolution I-GALFA (Inner-Galaxy ALFA) \schi\ 21-cm line survey data. The I-GALFA survey is a survey of the first Galactic quadrant visible to Arecibo ($\ell=32\arcdeg$ to 77\arcdeg\ and $|b| \la 15\arcdeg$) done by using the 7-beam Arecibo L-band Feed Array (ALFA) receiver on the Arecibo 305~m telescope, and it provides sensitive ($\Delta T_b=0.2$~K) and fully-sampled \schi\ maps at spatial and spectral resolutions of 4\arcmin\ and 0.184~\kms, respectively \citep{koo2010,gibson2012}. The I-GALFA survey data further reveal that there is a CHVC at the very center of GS040. This CHVC, named HVC 040+01$-$282 (hereafter CHVC040), was first identified in the Leiden/Dwingeloo survey \citep{wakker1991b} and was later classified as an isolated CHVC by \cite{braun1999} and \cite{deheij2002}. \citet{westmeier2005} presented a higher-resolution (9\arcmin) \schi\ image obtained from the Effelsberg telescope, which showed that CHVC040 has a pronounced head-tail structure. Our Arecibo \schi\ images reveal detailed spatial and velocity structure of CHVC040 strongly suggesting its association with the supershell GS040. We describe two structures in Section~\ref{sec:targets}, and discuss their physical characteristics and their association together with some implications on the disruption of HVCs in Section~\ref{sec:disc}. | \label{sec:disc} \subsection{Formation of GS040 by the Collision of CHVC040} \label{sec:disc_sub1} The location of CHVC040 at the geometrical center of GS040 suggests that their physical association is very likely. The centroids of the CHVC emission and that of the GS040's hub emission overlap within $\sim 0.2$~degrees. The probability of this being a random alignment is $\sim 3 \times 10^{-6}$, and multiplying this by $\sim 300$ CHVCs yields an overall probability of $9\times 10^{-4}$ for any CHVC aligning this well with the GS040's hub. No intermediate-velocity \schi\ connecting the two is apparent (Figure~\ref{fig:pvmap}), but this could be because the gas is ionized. We searched for a warm ionized gas associated with GS040 using the Wisconsin H$\alpha$ Mapper Northern Sky Survey (WHAM-NSS) data \citep{haffner2003}. The survey has an angular resolution of 1\arcdeg, and, around the GS040 area, it provides H$\alpha$ spectra covering $\vlsr$ from $-85$ to $+100$~\kms\ at spectral resolutions of 12~\kms. We have examined the H$\alpha$ intensity map integrated over the velocity range of GS040 ($-85$ to $-66$~\kms), but could not detect an associated emission ($\simlt$ 0.05~$R$ where $1 R = 10^6/4\pi\,{\rm photons\,cm}^{-2}\,{\rm sr}^{-1}\,{\rm s}^{-1}$). Note that this area is bright in the total H$\alpha$ intensity map with a mean intensity of about 3~$R$, so that we do not expect to see the faint emission associated with either GS040 or CHVC040 in the all-sky H$\alpha$ maps \citep{finkbeiner2003,dennison1998}. We also searched for an associated hot ionized gas using the 0.1-2.4 keV image of the ROSAT All-Sky X-ray Survey \citep[1 pixel scale = 44\arcsec;][]{voges1999}, but couldn't detect any emission. CHVC040 belongs to the ``Galactic Center Negative'' (GCN) HVC complex, which is a collection of small discrete HVCs sparsely distributed over a $70\arcdeg\times 70\arcdeg$ area within $\ell=0\arcdeg$ to $70\arcdeg$ and $b=-60\arcdeg$ to 10$\arcdeg$ \citep{wakker1991b,winkel2011}. A kinematic model has been proposed where GCN is a smooth gas flow starting at $b=-60\arcdeg$ at a heliocentric distance of 35~kpc and crossing the Galactic plane obliquely at 15~kpc \citep{jin2010}. For comparison, GS040 is probably located near the Scutum-Centaurus (Sct-Cen) arm at a distance of $\sim 20$~kpc \citep{dame2011} because the disrupted interstellar medium (ISM) is seen only at velocities below that of the Sct-Cen arm ($\sim -60$~\kms), not at higher velocities (see Figures~\ref{fig:chmap_gs040} and \ref{fig:pvmap}). We examined lists of known stellar objects, \schii\ regions, OB stars, and SN remnants (SNRs), but no known sources are likely to be associated with GS040. There is one \schii\ region (G$039.864+00.645$) in the {\it WISE} catalog of Galactic \schii\ regions \citep{anderson2014} that is located at $\sim 6\arcmin$ west of the hub. But this \schii\ region has a systematic velocity of $-40.9$~\kms\ \citep{anderson2011} and is enclosed by a small (68\arcsec) dust shell, so it cannot be responsible for the HI shell. Instead the distance of $\sim 20$~kpc is not unreasonable for CHVC040, because GCN does not have a smooth extended envelope like other HVC complexes, and it appears to be composed of several subpopulations that do not share a common origin \citep{winkel2011}. Note that the geometrical center of GS040 is well below the Galactic plane ($\sim 420\,\dtwenty$~pc where $\dtwenty\equiv d/20~{\rm kpc}$), i.e., at $b=+0\fdg6$ while the midplane there is at $b \sim +1\fdg8$ because the Galactic plane is warped in the outer Galaxy \citep{levine2006}. It is difficult to imagine the SN origin for a supershell at such height, and CHVC040 is most likely the energy/momentum source for GS040. The total energy deposited ($E_E$) in the Galactic disk by CHVC040 can be inferred from the parameters of the GS040 supershell. The radius of GS040 is $450\,\dtwenty$~pc, while its mass at $\vlsr \le -75$~\kms\ is $1.6\times 10^5\,\dtwenty^2$~\msol\ including the cosmic abundance of helium. If we account for the mass unobservable due to Galactic background \schi\ emission, the total mass of GS040 would be considerably greater. Adopting $v_s\sim 30$~\kms\ as the expansion speed of the shell, its kinetic energy is $E_K\ga 1.4\times 10^{51}\,\dtwenty^2$~erg. The collision should have occurred $\sim (1/3) R_s/v_s \sim 5\times 10^6\,\dtwenty$~yr ago, where the numerical factor $1/3$ accounts for the deceleration of the shell. Note that $E_K$ is a small fraction of the total energy deposited ($E_E$), most of which should have been radiated away. If GS040 was produced by multiple SNe, then, assuming instantaneous energy injection \citep{heiles1979}, $E_E\sim 5.3\times 10^{43}\, n_0^{1.12}\, R_s^{3.12}\, v_s^{1.4} \sim 1.2\times 10^{53}\, (n_0/0.1~{\rm cm}^{-3})$~erg, where $n_0$ is ambient hydrogen density. At the position of GS040, the mean \schi\ density in the midplane is $\sim 0.1\,{\rm cm}^{-3}$, and the \schi\ scale height is about 720~pc \citep{levine2006}. So $n_0\sim 0.06~{\rm cm}^{-3}$, and we have $E_E\sim 7\times 10^{52}$~erg. For comparison, the total extent of CHVC040 is $210\,\dtwenty\times 320\,\dtwenty\,{\rm pc}^2$ while its \schi\ mass is $\mchvc=5,\!800\,\dtwenty^2$~\msol. The area used to derive this mass has a geometrical mean radius of 150~pc, so that the mean hydrogen density of CHVC040 is 0.017~cm$^{-3}$. If CHVC040 collided with the rotating disk with the mean `deviation' speed (absolute difference in velocity from the disk gas there) of HVCs, i.e., $\sim 240$~\kms\ \citep{wakker2004}, the kinetic energy and momentum would be $4.7\times 10^{51}\,\dtwenty^2$~ergs and $2.0\times 10^{6}\,\dtwenty^2$~\msol\kms\ including the He abundance, respectively. This implies that the mass of CHVC040 when it collided with the disk should have been an order of magnitude greater and that the HVC that we see (CHVC040) might be the remains of the original HVC. The spatial morphology and velocity structure of CHVC040 suggest that it is moving southwest in the plane of the sky and is approaching us. The steep southwestern boundary of the cloud might represent the region compressed by the interaction with the ambient medium, whereas the diffuse envelope might be the material stripped off from the cloud due to the interaction or by the ram pressure of the surrounding medium \citep{santillan1999, kwak2009}. The small velocity width along the southwestern boundary and large velocity width beyond appear to be consistent with such speculation. Recently, \citet{heitsch2016} suggested that, for a CHVC with head-tail structure, the inclination angle, i.e., the angle between the CHVC's trajectory and the line-of-sight, can be derived from the asymmetry in position-velocity diagram along the head-tail line crossing the center of mass. The morphology of CHVC040 is different from typical head-tail CHVCs, i.e., CHVC040 has a wide flaring `tail' not a narrow elongated tail (see also \S~2), and its core is fragmented, so that it is not obvious if their model can be applied. Nevertheless if we assume that the center of mass is near the southwestern boundary and apply their model (their equations 1--4) to the position-velocity diagram along the red-dashed line in Figure 1, we obtain an inclination angle of $\sim 30\arcdeg$ which is consistent with our expectation. The shape of CHVC040 appears to be pointed away from the Galactic midplane as if it already went through the midplane. But the geometrical center of the supershell coincides with the current location of CHVC040, not being located in the midplane. Perhaps CHVC040 is approaching at an angle to the warped Galactic disk and has not yet fully penetrated the disk to the midplane. It is worth to note that, according to the \citet{jin2010}'s model, the GCN HVC stream is colliding to the Galactic plane almost perpendicularly {\em from below}, while the head-tail directions of individual HVCs seem to indicate that there is no preferential direction in their motions \citep{winkel2011}. Therefore, the orbit of CHVC040 is uncertain. The colliding geometry and the origin of the complex structures such as the hub and spokes in GS040 need to be explored. \subsection{CHVC040 and Disruption of HVCs} There are about three hundred known CHVCs in the Milky Way \citep{deheij2002, putman2002}. A considerable fraction of CHVCs has a head-tail structure indicating a ram pressure interaction with the diffuse galactic halo gas \citep{putman2011}. An important question is whether they are totally dissipated in the Galactic halo to feed the multi-phase circumgalactic medium or they can survive their trip through the halo \citep[e.g.,][]{putman2011}. Since CHVC040 is located in the far outer Galaxy, it may be of extragalactic origin rather than originating from a Galactic fountain, although it is not clear whether CHVC040 was originally a fragment of a nearby tidally disrupted galaxy or a cold cloud formed in a larger accreting flow of ionized, low-metallicity intergalactic gas. Our result then directly shows that at least some CHVCs of extragalactic origin do survive and collide with the Galactic disk. According to numerical studies, CHVCs with \schi\ masses $\la 3\times 10^4$~\msol\ would be totally disrupted in the Galactic halo unless they are embedded in dark matter \citep{heitsch2009, plockinger2012}. But dynamical shielding by an extended diffuse gaseous component can significantly extend their lifetime \citep{putman2012}. We have checked whether there are additional sources like the CHVC040-GS040 system in the I-GALFA \schi\ data using the HVC catalog of \cite{deheij2002}. There are twelve CHVCs in their Table~2 including CHVC040 in the I-GALFA survey area, most of which are at relatively high latitudes. They are isolated HVCs and have $\vlsr < -150$~\kms, so they belong to the GCN HVC complex. We see low-velocity \schi\ features around some CHVCs, but none of them appear associated. A systematic study against all CHVCs may reveal other CHVC-supershell systems. | 16 | 7 | 1607.07699 | Neutral atomic hydrogen (H I) gas in interstellar space is largely organized into filaments, loops, and shells, the most prominent of which are “supershells.” These gigantic structures, which require ≳ 3× {10}<SUP>52</SUP> erg to form, are generally thought to be produced by either the explosion of multiple supernovae (SNe) in OB associations or, alternatively, by the impact of high-velocity clouds (HVCs) falling into the Galactic disk. Here, we report the detection of a kiloparsec (kpc)-size supershell in the outskirts of the Milky Way with the compact HVC 040 + 01-282 (hereafter, CHVC040) at its geometrical center using the “Inner-Galaxy Arecibo L-band Feed Array” H I 21 cm survey data. The morphological and physical properties of both objects suggest that CHVC040, which is either a fragment of a nearby disrupted galaxy or a cloud that originated from an intergalactic accreting flow, collided with the disk ∼5 Myr ago to form the supershell. Our results show that some compact HVCs can survive their trip through the Galactic halo and inject energy and momentum into the Milky Way disk. | false | [
"supershells",
"data",
"the Milky Way disk",
"Galactic",
"OB associations",
"the Galactic disk",
"interstellar space",
"Feed Array” H I",
"energy",
"momentum",
"HVCs",
"HVC",
"high-velocity clouds",
"shells",
"loops",
"filaments",
"the disk",
"SNe",
"the Milky Way",
"an intergalactic accreting flow"
] | 11.293736 | 9.085638 | -1 |
4552757 | [
"Johansson, Jonas",
"Woods, Tyrone E.",
"Gilfanov, Marat",
"Sarzi, Marc",
"Chen, Yan-Mei",
"Oh, Kyuseok"
] | 2016MNRAS.461.4505J | [
"Diffuse gas in retired galaxies: nebular emission templates and constraints on the sources of ionization"
] | 28 | [
"Max-Planck Institut für Astrophysik, Karl-Schwarzschild-Str. 1, D-85741 Garching, Germany; Department of Biological and Environmental Sciences, University of Gothenburg, Medicinaregatan 18, SE-40530 Gothenburg, Sweden",
"Max-Planck Institut für Astrophysik, Karl-Schwarzschild-Str. 1, D-85741 Garching, Germany; Monash Centre for Astrophysics, School of Physics and Astronomy, 19 Rainforest Walk, Monash University, VIC 3800, Australia",
"Max-Planck Institut für Astrophysik, Karl-Schwarzschild-Str. 1, D-85741 Garching, Germany; Space Research Institute, Profsoyuznaya 84/32, 117997 Moscow, Russia; Kazan Federal University, Kremlevskaya str.18, 420008 Kazan, Russia",
"Centre for Astrophysics Research, University of Hertfordshire, Hatfield AL10 9AB, UK",
"Department of Astronomy, Nanjing University, Nanjing 210093, China; Key Laboratory of Modern Astronomy and Astrophysics (Nanjing University), Ministry of Education, Nanjing 210093, China",
"Department of Physics, Institute for Astronomy, ETH Zürich, Wolfgang-Pauli-Strasse 27, CH-8093 Zürich, Switzerland"
] | [
"2017ApJ...835...70Z",
"2017ApJ...837...40P",
"2017NatAs...1..800W",
"2018ApJ...854...18T",
"2018ApJ...854...52S",
"2018ApJ...854..105C",
"2018ApJ...863..120W",
"2018MNRAS.481..439W",
"2018MNRAS.481.1774H",
"2018MNRAS.481.4123K",
"2018RAA....18...49W",
"2018arXiv181007878C",
"2019AJ....158....2B",
"2019IAUS..343..371C",
"2019MNRAS.484L..79G",
"2019MNRAS.485.3169P",
"2019MNRAS.490.1678C",
"2019NewAR..8501523R",
"2020MNRAS.497.4981B",
"2021A&A...649A..63P",
"2021A&G....62.4.28B",
"2021ApJ...919...20M",
"2021IAUS..359..371V",
"2022EPJWC.26006002B",
"2022FrASS...9.3485S",
"2023A&G....64.5.37B",
"2023MNRAS.524.6295P",
"2024MNRAS.531..199O"
] | [
"astronomy"
] | 5 | [
"galaxies: elliptical and lenticular",
"cD",
"galaxies: ISM",
"Astrophysics - Astrophysics of Galaxies"
] | [
"1981PASP...93....5B",
"1984MNRAS.208..253M",
"1984PASP...96..287C",
"1986AJ.....91.1062P",
"1987ApJS...63..295V",
"1989ApJ...346..653K",
"1989S&T....78..491O",
"1994A&A...292...13B",
"1994A&AS..105..341G",
"1994ApJ...422..158O",
"1994ApJS...94..687W",
"1996A&AS..120..463M",
"1997AIPC..408..403C",
"1997AJ....113..162C",
"1998ApJ...500..525S",
"1999ApJ...513..861U",
"2000AJ....120.1579Y",
"2003ARA&A..41..391V",
"2003MNRAS.341...33K",
"2003MNRAS.344.1000B",
"2004ApJS..153....9G",
"2004PASP..116..138C",
"2005ApJ...619L...1M",
"2005ApJ...628..169S",
"2006MNRAS.366.1151S",
"2006MNRAS.366L...6P",
"2006MNRAS.371..703S",
"2007ApJS..173..267S",
"2007ApJS..173..619K",
"2007MNRAS.379..418C",
"2008MNRAS.391L..29S",
"2009ApJS..182..543A",
"2010AN....331..146R",
"2010MNRAS.402.2187S",
"2010Natur.463..924G",
"2011A&A...531A.109B",
"2011AJ....142..153Y",
"2011ApJS..195...13O",
"2011MNRAS.412.2183T",
"2011MNRAS.413.1687C",
"2012MNRAS.421..314C",
"2012MNRAS.421.1908J",
"2012PASA...29..447M",
"2013A&A...555L...1P",
"2013A&A...558A..43S",
"2013ApJ...764...41F",
"2013ApJ...771...62K",
"2013ApJS..208....7J",
"2013MNRAS.432.1640W",
"2014MNRAS.439.2351W",
"2014MNRAS.442.1079J",
"2014MNRAS.445.1912C",
"2015MNRAS.453.3024C"
] | [
"10.1093/mnras/stw1668",
"10.48550/arXiv.1607.02243"
] | 1607 | 1607.02243_arXiv.txt | 16 | 7 | 1607.02243 | We present emission-line templates for passively-evolving (`retired') galaxies, useful for investigation of the evolution of the interstellar medium in these galaxies, and characterization of their high-temperature source populations. The templates are based on high signal-to-noise (>800) co-added spectra (3700-6800 Å) of ∼11 500 gas-rich Sloan Digital Sky Survey galaxies devoid of star formation and active galactic nuclei. Stacked spectra are provided for the entire sample and sub-samples binned by mean stellar age. In our previous paper, Johansson et al., these spectra provided the first measurements of the He II 4686 Å line in passively-evolving galaxies, and the observed He II/Hβ ratio constrained the contribution of accreting white dwarfs (the `single-degenerate' scenario) to the Type Ia supernova rate. In this paper, the full range of unambiguously detected emission lines are presented. Comparison of the observed [O I] 6300 Å/Hα ratio with photoionization models further constrains any high-temperature single-degenerate scenario for Type Ia supernovae (with 1.5 ≲ T/10<SUP>5</SUP> K ≲ 10) to ≲3-6 per cent of the observed rate in the youngest age bin (I.e. highest SN Ia rate). Hence, for the same temperatures, in the presence of an ambient population of post-asymptotic giant branch stars, we exclude additional high-temperature sources with a combined ionizing luminosity of ≈1.35 × 10<SUP>30</SUP> L<SUB>⊙</SUB>/M<SUB>⊙,*</SUB> for stellar populations with mean ages of 1-4 Gyr. Furthermore, we investigate the extinction affecting both the stellar and nebular continuum. The latter shows about five times higher values. This contradicts isotropically distributed dust and gas that renders similar extinction values for both cases. | false | [
"stellar age",
"Type Ia",
"mean ages",
"post-asymptotic giant branch stars",
"stellar populations",
"active galactic nuclei",
"the Type Ia supernova rate",
"additional high-temperature sources",
"star formation",
"the youngest age bin",
"(I.e. highest SN Ia rate",
"SN",
"similar extinction values",
"accreting white dwarfs",
"their high-temperature source populations",
"the observed rate",
"SUP>5</SUP",
"∼11 500 gas-rich Sloan Digital Sky Survey galaxies",
"SUB>⊙</SUB>/M",
"any high-temperature single-degenerate scenario"
] | 12.711675 | 7.882671 | -1 |
||
12410206 | [
"Shirokov, S. I.",
"Lovyagin, N. Yu.",
"Baryshev, Yu. V.",
"Gorokhov, V. L."
] | 2016ARep...60..563S | [
"Large-scale fluctuations in the number density of galaxies in independent surveys of deep fields"
] | 8 | [
"Saint-Petersburg State University, St. Petersburg, Russia",
"Saint-Petersburg State University, St. Petersburg, Russia",
"Saint-Petersburg State University, St. Petersburg, Russia",
"Saint-Petersburg State University of Architecture and Civil Engineering, St. Petersburg, Russia"
] | [
"2017Ap.....60..484S",
"2020AstBu..75..207S",
"2020MNRAS.496.1530S",
"2020Univ....6..212B",
"2020Univ....6..215N",
"2021A&A...651A.114D",
"2021Univ....7..289R",
"2022Galax..10..108L"
] | [
"astronomy",
"physics"
] | 3 | [
"Astrophysics - Cosmology and Nongalactic Astrophysics",
"85A40",
"85A35"
] | [
"1996AJ....112.1335W",
"1996MNRAS.282..713K",
"2003IJMPD..12.1157T",
"2004ApJ...600L.171S",
"2005ApJ...624..463G",
"2005spcs.book.....G",
"2007ApJ...657..645P",
"2007ApJS..172..239M",
"2007ApJS..172..254G",
"2007Natur.445..286M",
"2008A&A...477..381S",
"2009A&A...505..463M",
"2009ApJ...690.1236I",
"2009AstBu..64..217L",
"2010ApJ...708..505K",
"2010ApJ...716..348B",
"2011A&A...535A..80M",
"2011ApJ...731..113M",
"2011CQGra..28p4003S",
"2012IJMPD..2130003K",
"2012MNRAS.419..556C",
"2013ApJS..206....8M",
"2013MNRAS.429.2910C",
"2014A&A...568A..46E",
"2014ApJ...782L...3C",
"2014MNRAS.441.2891M",
"2014Natur.513...71T"
] | [
"10.1134/S1063772916040107",
"10.48550/arXiv.1607.02596"
] | 1607 | 1607.02596_arXiv.txt | Modern observational cosmology has led to the discovery of very large structures with scales of order 100 Mpc in the spatial distribution of galaxies in the local Universe, at redshifts $z \sim 0.1$, and also in the spatial distribution of quasars at redshifts $z \sim 2$ $[1-6]$. Over the last decade, observations of the largescale structure of the Universe [7] have moved from groups and clusters of galaxies with sizes of the order of 1 Mpc to structures with sizes of $\sim 100$ Mpc (SDSS superclusters, in particular, the Sloan Great Wall, with a size of 420 Mpc [1]). The mass distribution can be determined independently from analyses of the proper motions of galaxies. Tully et al. [2] recently discovered a coherent motion of galaxies forming the Laniakea (Local) Supercluster with a diameter of 160 Mpc. Groups of quasars with scales of $10-100$ Mpc have also been found, beginning with the study of Komberg et al. [3, $4-6$]. Modern multi-band photometric deep galaxy surveys can be used to study the spatial distribution of galaxies at redshifts $0.3-3$, which has led to the detection of inhomogeneities on scales up to 1\,000 Mpc $[8-10]$. A comparison of the wideangle Sloan Digital Sky Survey (SDSS) and the COSMOS pencil-beam survey is shown in Fig. 1, together with the radial distribution of the number of galaxies. Fluctuations in the numbers of galaxies in neighboring volume elements of a pencil-beam survey are due to the presence of Poisson noise (the discreteness of the sample), systematic observational errors (selection effects), and the presence of large-scale structure (the "cosmic variance"), which plays an important role in comparisons of models with the observations. The main difficulty in distinguishing real density fluctuations is the possibility of hidden selection effects that are present in each galaxy survey, which can imitate large-scale inhomogeneity of the galaxy distribution. \begin{figure*} \centering \includegraphics[scale=0.35,clip]{Shirokov_fig1} \hfill \includegraphics[scale=0.35,clip]{Shirokov_fig2} \label{SDSS} \caption{ Upper: distribution of galaxies in the SDSS. The one-square-degree COSMOS deep field is marked by the dark strip. Lower: fit of the radial distribution of the number of galaxies using a uniform distribution. The gray area highlights regions where there are deficits or excesses of galaxies.} \end{figure*} In the current study, we present new arguments supporting the reality of large-scale fluctuations in the matter density in deep galaxy surveys. | Our analysis of the radial distribution of galaxies in the COSMOS/UVISTA field shows that the real observed fluctuations in the spatial distribution of the galaxies appreciably exceed the predictions of the $\Lambda$CDM model for the evolution of non-baryonic dark matter, in both their amplitude and linear size. This means that the $\Lambda$CDM model requires the introduction of a large bias factor ($b\sim10$ relative to the non-baryonic dark matter) at redshifts $z\sim1$. It is also necessary to explain the large scale of the positive correlation corresponding to the linear size of the detected structures. This follows from Table 3, which shows that the fluctuations in the number of galaxies preserve their sign over several adjacent bins, while neighboring bins should have opposite signs in the $\Lambda$CDM model. Thus, in addition to the difficulties of the $\Lambda$CDM model on small scales (galaxies and halos with sizes of $10-100$ kpc [28, 29]), there also exist problems on very large scales, associated with the presence of large-scale inhomogeneities in the spatial distribution of galaxies with sizes of the order of 1\,500 Mpc and amplitudes exceeding 20\%. \begin{figure} \centering \includegraphics[scale=0.66]{Shirokov_fig13} \caption{Comparison of $\delta_{obs}$ for the COSMOS (solid curve) and HDF-N (dotted curve) catalogs. The Poisson noise shown (dot-dashed curve) corresponds to $\sigma_{P}$ for the HDF-N catalog.} \label{HDF-N_COSMOS} \end{figure} Our analysis of the COSMOS and UVISTA survey data and comparisons of these data with the data from the ALHAMBRA, zCOSMOS, and XMMCOSMOS surveys leads to the following conclusions. \begin{itemize} \item The detected inhomogeneities in the radial distribution of galaxies in the COSMOS, UVISTA, ALHAMBRA, and XMMphot-COSMOS photometric catalogs is confirmed by data from the zCOSMOS and XMMspec-COSMOS spectroscopic catalogs, and these data are mutually consistent. \item The amplitudes and linear sizes of the fluctuations in the independent COSMOS (optical) and UltraVISTA (near infrared) catalogs, and also in the ALHAMBRA/Field 4, XMMNewton and zCOSMOS catalogs, are mutually consistent. The corresponding correlation coefficient is positive and equal to $\rho > 0.5$. \item The amplitudes and sizes of the fluctuations are stable for different fits and various limiting redshifts $z_{max}$. When the bin size is decreased, the fluctuation amplitude grows, and the individual density peaks coincide with galaxy clusters detected earlier. \end{itemize} The amplitudes and sizes we have found agree with the amplitudes and sizes of inhomogeneities found for the COSMOS field in other studies using the 10k-zCOSMOS spectral survey [22, 30], ALHAMBRA photometric survey [21], and X-ray observations [31]. Appreciable fluctuations in the number density of galaxies in slices of the COSMOS survey at various redshifts were found in [32], where is it emphasized that these structures really exist. Thirty-six candidate structures were found at redshifts $1.5 < z < 3.1$, having masses of $10^{15}M_{\odot}$. The sizes of the observed radial structures appreciably exceed the transverse cross sections of the pencil-beam surveys. The detected individual galaxy clusters fall near peaks of the fluctuations found using small redshift-bin sizes [25]. In particular, the peak at $z = 0.73$ corresponds to a galaxy cluster that was detected in [26] using spectroscopic observations, and the three peaks at $z\sim0.35$, $z\sim0.7$, and $z\sim0.85$ coincide with clusters detected in [22], as well as in our own study. The paper [21] describes the ALHAMBRA catalog, which includes the region of the COSMOS survey. This catalog also displays non-uniformity in the radial distribution of galaxies, which is correlated with the non-uniformity observed for the COSMOS survey. X-ray sources from the COSMOS catalog are considered in [31]. Peaks in the radial distribution of these X-ray sources agree with regions where there are excess galaxies in the optical and IR, indirectly supporting the presence of large-scale structures. The detection of fluctuations in the number of galaxies, manifest in the same way in independent observations and obtained using independent datareduction methods, substantially reduces the possibility that these are associated with unknown systematic errors. This suggests with a high degree of certainty that the fluctuations observed in the COSMOS/UVISTA field are related to the cosmic variance, and thus imply positive correlations in the spatial distribution of galaxies in deep surveys. \subsection* | 16 | 7 | 1607.02596 | New arguments supporting the reality of large-scale fluctuations in the density of the visible matter in deep galaxy surveys are presented. A statistical analysis of the radial distributions of galaxies in the COSMOS and HDF-N deep fields is presented. Independent spectral and photometric surveys exist for each field, carried out in different wavelength ranges and using different observing methods. Catalogs of photometric redshifts in the optical (COSMOS-Zphot) and infrared (UltraVISTA) were used for the COSMOS field in the redshift interval 0.1 < z < 3.5, as well as the zCOSMOS (10kZ) spectroscopic survey and the XMM-COSMOS and ALHAMBRA-F4 photometric redshift surveys. The HDFN-Zphot and ALHAMBRA-F5 catalogs of photometric redshifts were used for the HDF-N field. The Pearson correlation coefficient for the fluctuations in the numbers of galaxies obtained for independent surveys of the same deep field reaches R = 0.70 ± 0.16. The presence of this positive correlation supports the reality of fluctuations in the density of visible matter with sizes of up to 1000 Mpc and amplitudes of up to 20% at redshifts z ~ 2. The absence of correlations between the fluctuations in different fields (the correlation coefficient between COSMOS and HDF-N is R = -0.20 ± 0.31) testifies to the independence of structures visible in different directions on the celestial sphere. This also indicates an absence of any influence from universal systematic errors (such as "spectral voids"), which could imitate the detection of correlated structures. | false | [
"different fields",
"deep galaxy surveys",
"different directions",
"different observing methods",
"different wavelength ranges",
"R = -0.20 ±",
"photometric redshifts",
"correlated structures",
"visible matter",
"independent surveys",
"HDF-N",
"COSMOS",
"structures",
"R =",
"redshifts",
"N",
"lt",
"the COSMOS field",
"the same deep field",
"z"
] | 11.750803 | 3.459156 | -1 |